Most of the rock we see around us, from the ocean floor to the tallest mountains of the Himalayas, is no more than a few hundred million years old. The oldest oceanic rock is about 230 million years old, for example, and little continental rock is more than a couple of billion years old [Poupinet and Shapiro, 2009]. However, we know that Earth is much older, in part because we know that rocky relics of much older eras survive within the interiors of today’s continents.
None of the rock on Earth’s surface dates back to the planet’s earliest days, but detailed studies of isotopic decay in minerals from Earth’s mantle and from meteorites and lunar samples have produced a consensus that the planet is 4.54 billion years old, give or take 50 million years, at least. (Material from the oldest known meteorites dates back about 4.57 billion years, so we know that our solar system—and possibly Earth as well—is at least this old.)
Plate tectonics, coupled with convection in the mantle, governs the destructive forces applied to Earth’s lithosphere, its topmost layer of rock. Whereas oceanic lithosphere is recycled in subduction zones after a couple of hundred million years or so, continental lithosphere often survives longer but is still ultimately deformed, eroded, and destroyed by forces exerted by the underlying convective mantle. Thus, on a tectonically active Earth, rocks older than a few billion years should be rare to nearly nonexistent.
However, such ancient rock, dating back more than 3 billion years (and perhaps even more than 4 billion years), has been found in different parts of the world (Figure 1). These very old rocks are known as cratons, from the Greek root word κράτος, meaning strength. Understanding how cratons have survived for such a long time, some almost since the birth of the planet, remains one of the grand challenges of geodynamics.
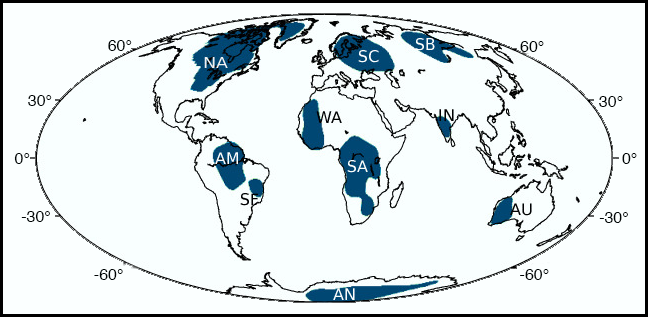
Rise, Melt, and Squeeze
Not only do we not understand fully how cratons have managed to survive to the present day, but also there is no consensus on the mechanism that formed the thick, old formations of cratonic lithosphere in the first place. Geochemical and geophysical studies have shown that cratonic lithosphere is at least twice as thick as the surrounding lithosphere, which is typically about 100 kilometers thick.
Many studies have suggested that hot upwellings (e.g., mantle plumes) come from deep inside Earth (Figure 2a) and that they might have led to the formation of cratons [Lee et al., 2011]. These upwellings produce large quantities of magma, both from the deep Earth and from shallower surrounding rock incorporated into the upwelling as it rises to the surface (Figure 2b). Upon cooling, this melt can create new solid lithosphere (Figure 2c). Indeed, Earth’s interior was still a few hundred degrees hotter than the present day when cratons were forming. Such hot upwellings or mantle plumes likely occurred more often on early Earth than they do today and thus could have formed large landmasses.
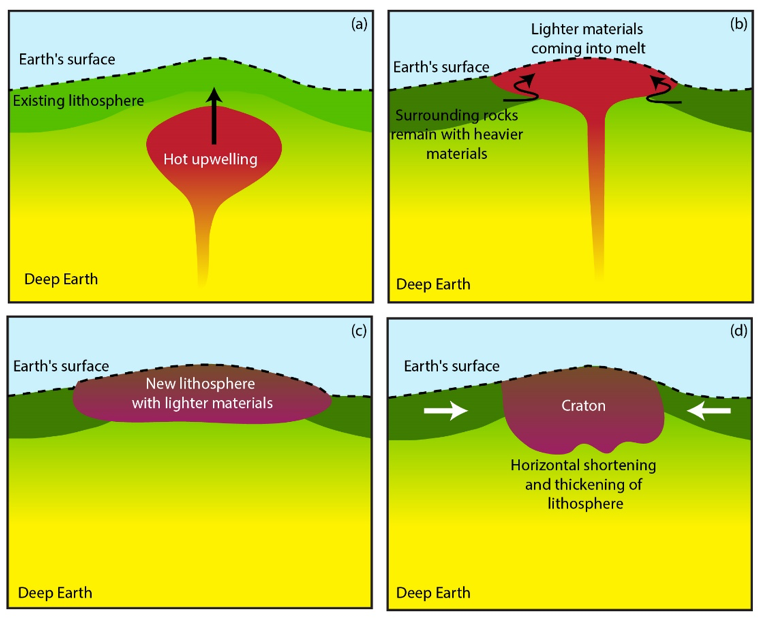
However, for the newly formed lithosphere to have become so much thicker than the surrounding lithosphere, some kind of compressional, or shortening, process might have been needed (Figure 2d) [Wang et al., 2018]. Exactly how this shortening took place is not yet clear because Earth’s present mode of tectonism, the plate tectonics that creates shortening or extension of the surface, might not have been fully active roughly 3 billion years ago when cratons were forming. Although the exact mechanisms are uncertain, however, we know there are at least two crucial factors required for craton formation: a large quantity of melt rising to the surface and a shortening process to thicken the lithosphere.
Long Live the Cratons
Even if these craton formation hypotheses prove satisfying, questions about why cratons still exist remain. Over the past billion or so years, tectonic processes have subjected other regions of Earth’s lithosphere to multiple rounds of destruction and re-creation, so how have cratons survived?
The reasons are closely linked to their formation process. Chemical buoyancy was one of the first proposed explanations [Jordan, 1975, 1978]. This explanation rests on observations from geochemical studies that cratons are composed primarily of rock that is less dense than the surrounding rock and thus can be said to float amid their surroundings.
Buoyancy alone is insufficient for craton survival against tectonic recycling; cratonic material must also be strong enough to resist recycling.
As the large quantities of hot, craton-forming melt rose through the mantle and existing lithosphere en route to the surface, they could have become enriched in lighter minerals as heavier minerals crystallized and remained deeper below the cratonic lithosphere (Figure 2b). When the melt solidified, it formed new lithosphere preferentially made of the lighter materials (Figure 2c) and thus maintained its freeboard above denser mantle rock.
Physically testing this hypothesis after it was proposed remained a challenge because scientists rarely have direct access to rock samples from the deep Earth. With advances in computing, numerical modeling gradually became an important tool for studying Earth’s deep interior. The first numerical test of Jordan’s hypothesis about cratonic survival was published in 1999 [Lenardic and Moresi, 1999]. This study showed that buoyancy alone is insufficient for craton survival against tectonic recycling and that cratonic material must also be strong enough to resist recycling.
Viscosity, a material’s resistance to flowing, plays an important role in understanding a material’s strength. Research suggests not only that cratonic material is relatively light but also that it is highly viscous [Lenardic et al., 2003]. For comparison, the viscosity of liquid water is roughly 10−2 pascal seconds; for honey, it is about 10 pascal seconds; and a glacier’s viscosity is on the order of 1012 (1 trillion) pascal seconds. The viscosity of the upper mantle surrounding cratons has been estimated to be about 1021 pascal seconds: 1 billion times more viscous than a glacier. And modeling suggests that cratons are at least 100–1,000 times more viscous than their surroundings, likely helping them resist tectonic recycling [Paul and Ghosh, 2020].
Survival of the Strongest
Recent numerical models representing the evolution of Earth’s cratons over roughly the past 400 million years suggest that strong, highly viscous cratons survive over such long periods, whereas weak cratons are destroyed [Paul and Ghosh, 2020]. The video clips below show two simulations from this study. The first clip shows a craton composed of relatively weaker, less viscous material, and the second clip shows a craton composed of stronger, more viscous material.
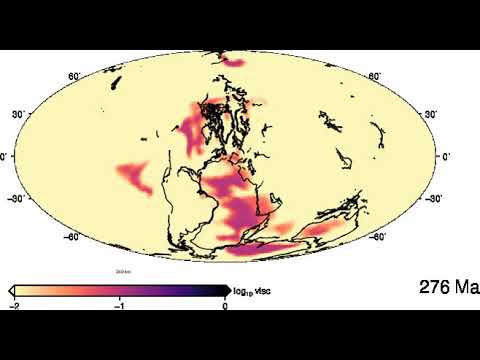

The viscosity of a material depends partly on its fluid content. Cratons could have attained their high viscosity during their formation and evolution because large quantities of melt retain heat longer, and thus, fluids have more time to escape from the melt. Also, during periods of horizontal shortening (Figure 2d), fluids can be squeezed from newly formed rock in a process called dehydration.
A craton’s thickness can also stabilize it. Numerical studies show that more viscous, thicker cratons can resist tectonic forces more efficiently [Paul et al., 2019]. Some of the thickest cratonic lithosphere is the most difficult to recycle.
Thus, we find that cratonic lithosphere has three qualities that distinguish it from other lithosphere. Cratons are made up of lighter (chemically buoyant) materials, they are highly viscous (100–1,000 times more than surrounding rock), and they are almost twice as thick as younger lithosphere. With these conditions working in their favor, it makes sense that cratons have remained stable over billions of years.
Yet not all cratons have remained stable since their birth. Parts of the North China craton and the North American craton have reportedly been destroyed [e.g., Zhu et al., 2012], with previous studies citing multiple hypotheses as to why this might have occurred [e.g., Lee et al., 2011].
One of the most well-known hypotheses is that just as dehydration strengthens cratonic lithosphere, the addition of fluid (water) into cratonic lithosphere can weaken it. This process of adding water into the cratonic lithosphere, called metasomatism, can occur if a craton encounters another mantle plume or water-rich rock body that releases water into the craton. The water reacts with and chemically alters the cratonic rock, thereby decreasing its strength. A weakened craton is then more prone to tectonic recycling.
A Record in the Rock
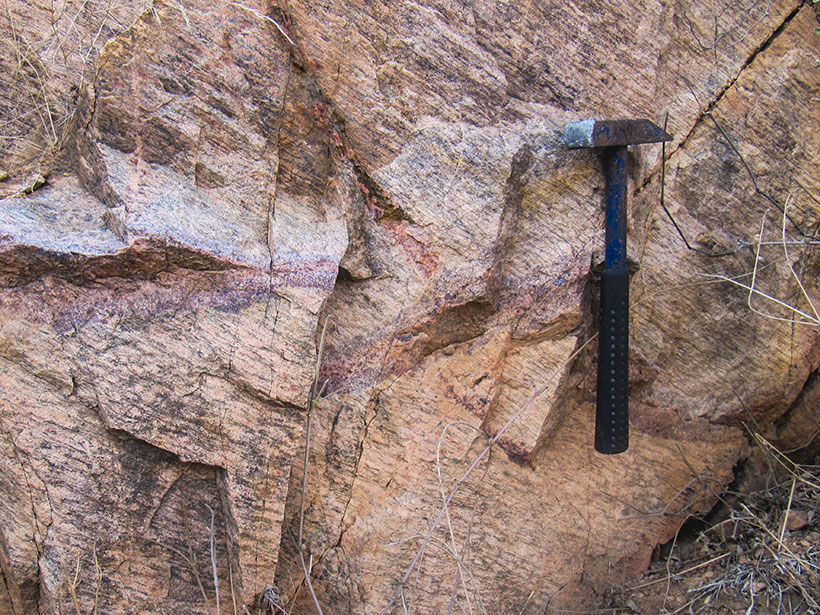
Beyond satisfying scientific curiosity, craton studies explore larger questions about Earth’s planetary evolution and its uniqueness in the solar system. Rock samples from Earth’s infancy more than 4.5 billion years ago would help answer these questions, but unfortunately, these samples are unavailable. Neither is it possible to simulate Earth in a laboratory experiment that runs for billions of years. But cratons are like natural museums that preserve evidence of changes in physical and chemical conditions during much of Earth’s evolution. Deciphering this evidence reveals information about the origins of plate tectonics, which are closely related to craton formation, as well as about the conditions that favor life on the planet.
Today Earth’s lithosphere takes part in the large-scale mantle convection that gradually circulates hot material from deep inside the planet toward its surface and carries cold material from the surface back into the deep Earth (Figure 3, left). By contrast, in early Earth, mantle materials circulated beneath an intact lithosphere (Figure 3, right). Exactly how and when the mode of tectonism transformed from the older “stagnant lid” tectonics to the present mobile lid (or simply “plate”) tectonics have remained enigmatic and are among the major challenges of geodynamics.
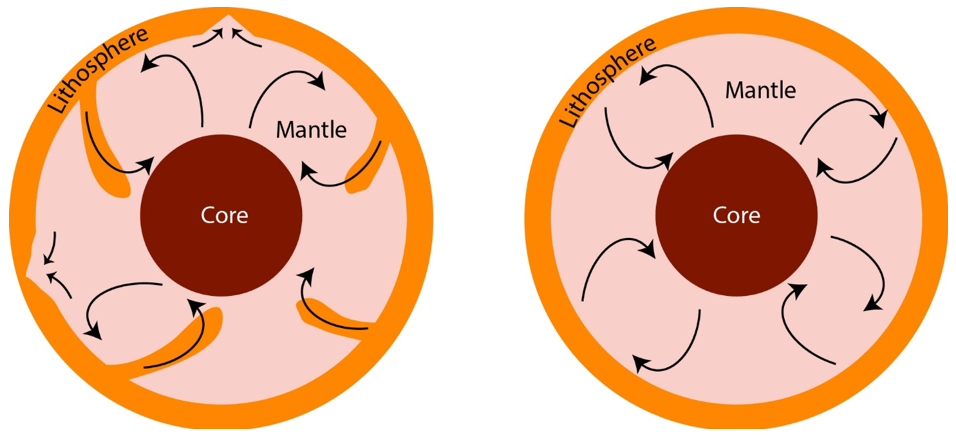
The onset of plate tectonics might have caused a rapid change in the chemistry of magma from mafic (rich in magnesium and iron) to more felsic (rich in lighter components like feldspar and silica) [Tang et al., 2016], producing the lighter and more viscous rock that is the primary building block of cratons. This change in tectonic mode could also have generated massive compressive forces that might have triggered lithospheric shortening and initial thickening of cratons [Beall et al., 2018].
Over many millions of years, these cratons have chemically weathered under the atmosphere. Weathering of felsic silicate rock removes an estimated 150–330 million tons of carbon dioxide from the atmosphere each year. This mechanism increased the relative proportion of oxygen in the atmosphere, leading to global climate change and contributing to the conditions necessary for life to flourish on the planet.
Cratons are the only rocks remaining from the terrestrial distant past, and they link this past to the present. Understanding how they evolved and survived refines our knowledge about Earth’s evolution and how our home turned out so differently than other rocky planets in the solar system.
Acknowledgments
Numerical models were conducted at Supercomputer Education and Research Centre, Indian Institute of Science, Bangalore.
References
Beall, A. P., L. Moresi, and C. M. Cooper (2018), Formation of cratonic lithosphere during the initiation of plate tectonics, Geology, 46(6), 487–490, https://doi.org/10.1130/G39943.1.
Jordan, T. H. (1975), The continental tectosphere, Rev. Geophys., 13(3), 1–12, https://doi.org/10.1029/RG013i003p00001.
Jordan, T. H. (1978), Composition and development of the continental tectosphere, Nature, 274(5671), 544–548, https://doi.org/10.1038/274544a0.
Lee, C.-T. A., P. Luffi, and E. J. Chin (2011), Building and destroying continental mantle, Annu. Rev. Earth Planet. Sci., 39, 59–90, https://doi.org/10.1146/annurev-earth-040610-133505.
Lenardic, A., and L.-N. Moresi (1999), Some thoughts on the stability of cratonic lithosphere: Effects of buoyancy and viscosity, J. Geophys. Res., 104(B6), 12,747–12,758, https://doi.org/10.1029/1999JB900035.
Lenardic, A., L.-N. Moresi, and H. Mühlhaus (2003), Longevity and stability of cratonic lithosphere: Insights from numerical simulations of coupled mantle convection and continental tectonics, J. Geophys. Res., 108(B6), 2303, https://doi.org/10.1029/2002JB001859.
Paul, J., and A. Ghosh (2020), Evolution of cratons through the ages: A time-dependent study, Earth Planet. Sci. Lett., 531, 115962, https://doi.org/10.1016/j.epsl.2019.115962.
Paul, J., A. Ghosh, and C. P. Conrad (2019), Traction and strain-rate at the base of the lithosphere: An insight into cratonic stability, Geophys. J. Int., 217(2), 1,024–1,033, https://doi.org/10.1093/gji/ggz079.
Poupinet, G., and N. M. Shapiro (2009), Worldwide distribution of ages of the continental lithosphere derived from a global seismic tomographic model, Lithos, 109(1–2), 125–130, https://doi.org/10.1016/j.lithos.2008.10.023.
Tang, M., K. Chen, and R. L. Rudnick (2016), Archean upper crust transition from mafic to felsic marks the onset of plate tectonics, Science, 351(6271), 372–375, https://doi.org/10.1126/science.aad5513.
Wang, H., J. van Hunen, and D. G. Pearson (2018), Making Archean cratonic roots by lateral compression: A two-stage thickening and stabilization model, Tectonophysics, 746, 562–571, https://doi.org/10.1016/j.tecto.2016.12.001.
Zhu, R., et al. (2012), Destruction of the North China craton, Sci. China Earth Sci., 55(10), 1,565–1,587, https://doi.org/10.1007/s11430-012-4516-y.
Author Information
Jyotirmoy Paul ([email protected]), Indian Institute of Science, Bengaluru, Karnataka, India
Citation:
Paul, J. (2021), Cratons, why are you still here?, Eos, 102, https://doi.org/10.1029/2021EO156381. Published on 25 March 2021.
Text © 2021. The authors. CC BY-NC-ND 3.0
Except where otherwise noted, images are subject to copyright. Any reuse without express permission from the copyright owner is prohibited.