The evolution and habitability of Earth and other worlds are largely products of how much these worlds are warmed by their parent stars, by the decay of radioactive elements in their interiors, and by other external and internal processes.
Of these processes, tidal heating caused by gravitational interactions among nearby stars, planets, and moons is key to the way that many worlds across our solar system and beyond have developed. Jupiter’s intensely heated moon Io, for example, experiences voluminous lava eruptions like those associated with mass extinctions on ancient Earth, courtesy of tidal heating. Meanwhile, less intense tidal heating of icy worlds sometimes maintains subsurface oceans—thought to be the case on Saturn’s moon Enceladus and elsewhere—greatly expanding the habitable zones around stars.
Tidal heating results from the changing gravitational attraction between a parent planet and a close-in moon that revolves around that planet in a noncircular orbit. (The same goes for planets in close noncircular orbits around parent stars.) Because its orbit is not circular, the distance between such a moon and its parent planet varies depending on where it is in its orbit, which means it experiences stronger or weaker gravitational attraction to its parent body at different times. These tightening and relaxing responses of the gravitational attraction change the orbiting moon’s shape over the course of each orbit and generate friction and heat internally as rock, ice, and viscous magma are pushed and pulled. (The same process causes Earth’s ocean tides, although the reshaping of the ocean generates relatively little heat because of water’s low viscosity.)
Tidal deformation is thus central to understanding a moon’s energy budget and probing its internal structure.
The magnitude and phase of a moon’s tidally induced deformation depend on its interior structure. Bodies with continuous liquid regions below the surface, such as a subsurface water or magma ocean, are expected to show larger tidal responses and perhaps distinctive rotational parameters compared with bodies without these large fluid regions. Tidal deformation is thus central to understanding a moon’s energy budget and probing its internal structure.
The dissipation of tidal energy (or the conversion of orbital energy into heat) within a parent planet causes the planet’s moons to migrate outward. This process frequently drives the satellites into what are called mean-motion resonances with each other, in which their orbital periods—the time it takes a satellite to complete a revolution around its parent—are related by integer ratios. The multiple satellites within such orbital resonances exert periodic gravitational influences on each other that serve to maintain noncircular orbits (orbits with nonzero eccentricities), which drive tidal heating. Simultaneously, tidal dissipation and heating within orbiting satellites damp the orbital eccentricity excited by mean-motion resonances, move orbits inward, and power tectonism and potential volcanic activity. Without resonances, continued tidal energy dissipation would eventually lead to circular orbits that would minimize tidal heating.
For as much as we know, there remain fundamental gaps in our understanding of tidal heating. At a Keck Institute for Space Studies workshop [de Kleer et al., 2019] in October 2018, participants discussed the current state of knowledge about tidal heating as well as how future spacecraft missions to select solar system targets could help address these gaps.
Jupiter and the Galilean Satellites
Each time Ganymede orbits Jupiter once, Europa completes two orbits, and Io completes four orbits (Figure 1). This 1:2:4 resonance was discovered by Pierre-Simon Laplace in 1771, but its significance was realized only 200 years later when Peale et al. [1979] published their prediction that the resonance would lead to tidal heating and melting of Io, just before the Voyager 1 mission discovered Io’s active volcanism. The periodic alignment of these three large moons results in forced eccentric orbits, so the shapes of these moons periodically change as they orbit massive Jupiter, with the most intense deformation and heating occurring at innermost Io. Meanwhile, tidal heating of Europa (and of Saturn’s moon Enceladus) maintains a subsurface ocean that’s below a relatively thin ice shell and in contact with the moon’s silicate core, providing key ingredients for habitability.
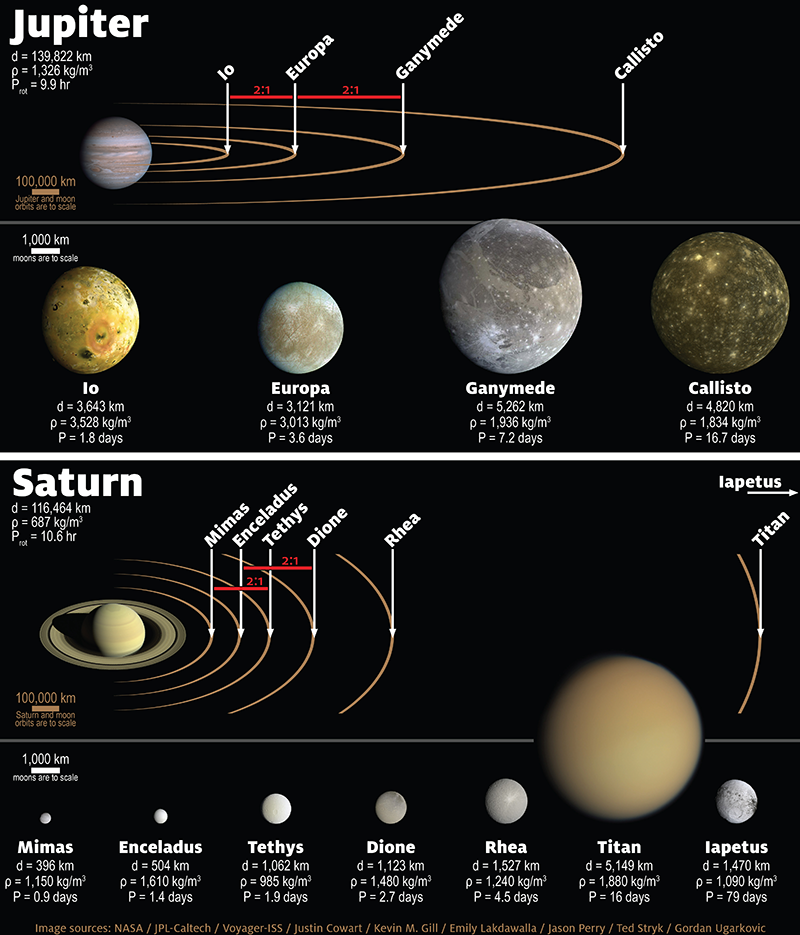
Although Peale et al. [1979] predicted the presence of a thin lithosphere over a magma ocean on Io, Voyager 1 revealed mountains more than 10 kilometers high (Figure 2). This suggests that Io has a thick, cold lithosphere formed by rapid volcanic resurfacing and subsidence of crustal layers. The idea of a magma ocean inside Io generally lost favor in subsequent studies, until Khurana et al. [2011] presented evidence from Galileo mission data of an induced magnetic signature from Io. Induced signatures from Europa, Ganymede, and Callisto (another of Jupiter’s moons that with Io, Europa, and Ganymede, make up what are known as the Galilean satellites) had previously been interpreted as being caused by salty oceans, which are electrically conducting—and molten silicates are also electrically conducting. Considerable debate persists about whether Io has a magma ocean.
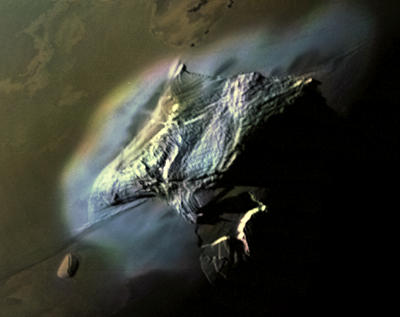
The Jovian system provides the greatest potential for advances in our understanding of tidal heating in the next few decades. This is because NASA’s Europa Clipper and the European Space Agency’s Jupiter Icy Moons Explorer (JUICE) will provide in-depth studies of Europa and Ganymede in the 2030s, and the Juno mission orbiting Jupiter may have close encounters with the Galilean satellites in an extended mission. However, our understanding of this system will continue to be limited unless there is also a dedicated mission with close encounters of Io. The easily observed heat flow on Io (at least 20 times greater than that on Earth) from hundreds of continually erupting volcanoes makes it the ideal target for further investigation and key to understanding the Laplace resonance and tidal heating.
Advances from the Saturnian System
As discovered by Hermann Struve in 1890, the Saturnian system contains two pairs of satellites that each display 1:2 orbital resonance (Figure 1): Tethys-Mimas and Dione-Enceladus. More recently, the Cassini mission discovered that Enceladus and Titan are ocean worlds, hosting large bodies of liquid water beneath icy crusts.
Precise measurements of the Saturnian moon orbits, largely based on Cassini radio tracking during close encounters, have revealed outward migration rates much faster than expected. But extrapolating the Cassini migration measurement backward in time while using the conventional assumption of a constant tidal dissipation parameter Q, which measures a body’s response to tidal distortion, implies that the Saturnian moons would have, impossibly, been inside Saturn in far less time than the lifetime of the solar system. To resolve this contradiction, Fuller et al. [2016] proposed a new theory for tidally excited systems that describes how orbital migrations could accelerate over time.
The theory is based on the idea that the internal structures of gas giant planets can evolve on timescales comparable to their ages, causing the frequencies of a planetary oscillation mode (i.e., the planet’s vibrations) to gradually change. This evolution enables “resonance locking” in which a planetary oscillation mode stays nearly resonant with the forcing created by a moon’s orbital period, producing outward migration of the moon that occurs over a timescale comparable to the age of the solar system. This model predicts similar migration timescales but different Q values for each moon. Among other results, this hypothesis explains the present-day heat flux of Enceladus without requiring it to have formed recently, a point relevant to its current habitability and a source of debate among researchers.
Observing Tidally Heated Exoplanets
Beyond our solar system, tidal heating of exoplanets and their satellites significantly enlarges the total habitable volume in the galaxy. And as exoplanets continue to be confirmed, researchers are increasingly studying the process in distant star systems. For example, seven roughly Earth-sized planets orbit close to TRAPPIST-1 (Figure 3), a low-mass star about 40 light-years from us, with periods of a few Earth days and with nonzero eccentricities. Barr et al. [2018] concluded that two of these planets undergo sufficient tidal heating to support magma oceans and the other five could maintain water oceans.
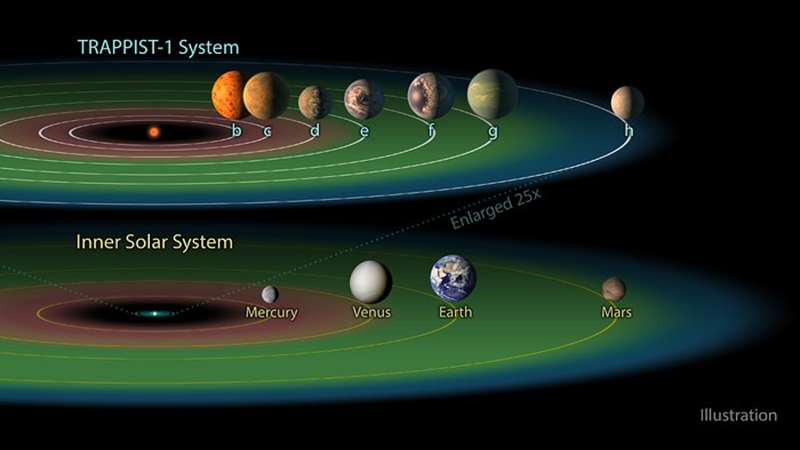
Highly volcanic exoplanets are considered high-priority targets for future investigations because they likely exhibit diverse compositions and volcanic eruption styles. They are also relatively easy to characterize because of how readily volcanic gases can be studied with spectroscopy, their bright flux in the infrared spectrum, and their preferential occurrence in short orbital periods. The latter point means that they can be observed relatively often as they frequently transit their parent stars, resulting in a periodic slight dimming of the starlight.
Directions in Tidal Heating Research
The Keck Institute of Space Studies workshop identified five key questions to drive future research and exploration:
Properties of the erupted material can place strong constraints on the temperature and viscosity structure of a planet with depth.
1. What do volcanic eruptions tell us about planetary interiors? Active eruptions in the outer solar system are found on Io and Enceladus, and there are suggestions of such activity on Europa and on Neptune’s large moon Triton. Volcanism is especially important for the study of planetary interiors, as it provides samples from depth and shows that there is sufficient internal energy to melt the interior. Eruption styles place important constraints on the density and stress distribution in the subsurface. And for tidally heated bodies, the properties of the erupted material can place strong constraints on the temperature and viscosity structure of a planet with depth, which is critical information for modeling the distribution and extent of tidal dissipation.
2. How is tidal dissipation partitioned between solid and liquid materials? Tidal energy can be dissipated as heat in both the solid and liquid regions of a body. The dissipation response of planetary materials depends on their microstructural characteristics, such as grain size and melt distribution, as well as on the timescales of forcing. If forcing occurs at high frequency, planetary materials respond via instantaneous elastic deformation. If forcing occurs at very low frequency, in a quasi steady state manner, materials respond with permanent viscous deformation. Between these ends of the spectrum, on timescales most relevant to tidal flexing of planetary materials, the response is anelastic, with a time lag between an applied stress and the resulting deformation.
Decades of experimental studies have focused on studying seismic wave attenuation here on Earth. However, seismic waves have much smaller stress amplitudes and much higher frequencies than tidal forcing, so the type of forcing relevant to tidally heated worlds remains poorly explored experimentally. For instance, it is not clear under what conditions tidal stress could alter existing grain sizes and/or melt distributions within the material being stressed.
3. Does Io have a magma ocean? To understand Io’s dynamics, such as where tidal heating occurs in the interior, we need to better understand its interior structure. Observations collected during close spacecraft flybys can determine whether Io has a magma ocean or another melt distribution (Table 1 and Figure 4). One means to study this is from magnetic measurements. Such measurements would be similar to the magnetic field measurements made by the Galileo spacecraft near Io but with better data on Io’s plasma environment (which is a major source of noise), flybys optimized to the best times and places for measuring variations in the magnetic field, and new laboratory measurements of electrical conductivities of relevant planetary materials.
If there is a continuous liquid layer within Io and the overlying lithosphere is rigid, libration amplitudes could be easily measurable with repeat images taken by a spacecraft.
A second method to investigate Io’s interior is with gravity science, in which the variables k2 and h2 (Table 1), called Love numbers, express how a body’s gravitational potential responds on a tidal timescale and its radial surface deformation, respectively. Each of these variables alone can confirm or reject the hypothesis of a liquid layer decoupled from the lithosphere because their values are roughly 5 times larger for a liquid than a solid body. Although k2 can be measured through radio science (every spacecraft carries a radio telecommunication system capable of this), the measurement of h2 requires an altimeter or high-resolution camera as well as good knowledge of the spacecraft’s position in orbit and orientation.
Libration amplitude provides an independent test for a detached lithosphere. The orbit of Io is eccentric, which causes its orbital speed to vary as it goes around Jupiter. Its rotational speed, on the other hand, is nearly uniform. Therefore, as seen from Jupiter, Io appears to wobble backward and forward, as the Moon does from the vantage of Earth. Longitudinal libration arises in Io’s orbit because of the torque applied by Jupiter on Io’s static tidal and rotational bulge while it is misaligned with the direction toward Jupiter. If there is a continuous liquid layer within Io and the overlying lithosphere is rigid (as is thought to be needed to support tall mountains), libration amplitudes greater than 500 meters are expected—a scale easily measurable with repeat images taken by a spacecraft.
Table 1. Testing Models for Tidal Heating and Melt Distribution in Io
Model | Tidal k2 or h2 | Libration Amplitude | Magnetic Induction | Major Lava | Heat Flow |
---|---|---|---|---|---|
I | low | small | weak | high-temperature basaltic | more polar |
II | low | small | weak | basaltic | more equatorial |
III | high | large | strong | very high temperature ultramafic | equatorial or uniform |
IV | low | small | strong | very high temperature ultramafic | equatorial or uniform |
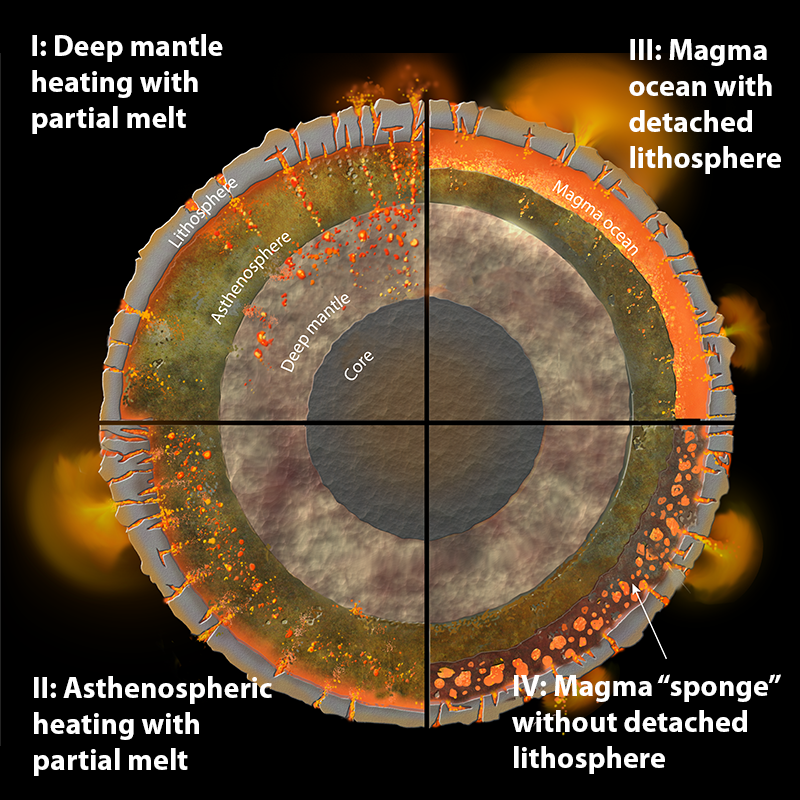
4. Is Jupiter’s Laplace system in equilibrium? The Io-Europa-Ganymede system is a complex tidal engine that powers Io’s extreme volcanism and warms Europa’s water ocean. Ultimately, Jupiter’s rotational energy is converted into a combination of gravitational potential energy (in the orbits of the satellites) and heat via dissipation in both the planet and its satellites. However, we do not know whether this system is currently in equilibrium or whether tidal migration and heating rates and volcanic activity vary over time.
The orbital evolution of the system can be determined from observing the positions of the Galilean satellites over time. A way of verifying that the system is in equilibrium is to measure the rate of change of the semimajor axis for the three moons in the Laplace resonance. If the system is in equilibrium, the tidal migration timescale must be identical for all three moons. Stability of the Laplace resonance implies a specific equilibrium between energy exchanges in the whole Jovian system and has implications for its past and future evolution.
5. Can stable isotopes inform our understanding of the long-term evolution of tidal heating? We lack knowledge about the long-term evolution of tidally heated systems, in part because their geologic activity destroys the older geologic record. Isotopic ratios, which preserve long-term records of processes, provide a potential window into these histories. If processes like volcanic eruptions and volatile loss lead to the preferential loss of certain isotopes from a moon or planet, significant fractionation of a species may occur over the age of the solar system. However, to draw robust conclusions, we must understand the current and past processes that affect the fractionation of these species (Figure 5), as well as the primordial isotopic ratios from the body of interest. Measurements of isotopic mass ratios—in, for example, the atmospheres and volcanic plumes of moons or planets of interest—in combination with a better understanding of these fractionation processes can inform long-term evolution.
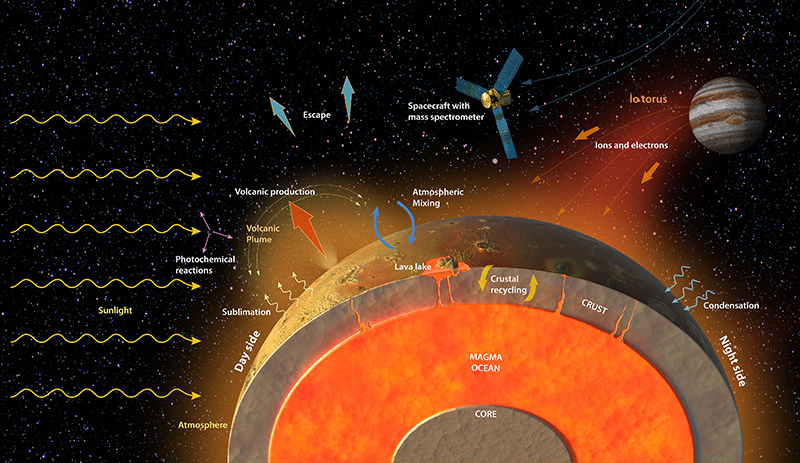
Missions to the Moons
Both the Europa Clipper and JUICE are currently in development and are expected to arrive at Jupiter in the late 2020s or early 2030s. One of the most important measurements made during these missions could be precision ranging during close flybys to detect changes in the orbits of Europa, Ganymede, and Callisto, which would provide a key constraint on equilibrium of the Jovian system if we can acquire comparable measurements of Io. JUICE will be the first spacecraft to orbit a satellite (Ganymede), providing excellent gravity, topography, magnetic induction, and mass spectrometry measurements.
The most promising avenue to address the five key questions is a new spacecraft mission that would make multiple close flybys of Io combined with laboratory experiments and Earth-based telescopic observations.
The Dragonfly mission to Titan includes a seismometer and electrodes on the landing skids to sense electric fields that may probe the depth to Titan’s interior water ocean. Potential Europa and Enceladus landers could also host seismometers. The ice giants Uranus and Neptune may also finally get a dedicated mission in the next decade. The Uranian system contains six medium-sized moons and may provide another test of the resonance locking hypothesis suggested by Fuller et al. [2016], and Neptune’s active moon Triton is another strong candidate to host an ocean.
The most promising avenue to address the five key questions noted at the Keck Institute of Space Studies workshop is a new spacecraft mission that would make multiple close flybys of Io [McEwen et al., 2019], combined with laboratory experiments and Earth-based telescopic observations. An Io mission could characterize volcanic processes to address question 1, test interior models via geophysical measurements coupled with laboratory experiments and theory to address questions 2 and 3, measure the rate of Io’s orbital migration to determine whether the Laplace resonance is in equilibrium to address question 4, and determine neutral compositions and measure stable isotopes in Io’s atmosphere and plumes to address question 5.
Acknowledgments
We thank Michelle Judd and others at the Keck Institute for Space Studies and all participants in the tidal heating study. Part of the research was carried out at the Jet Propulsion Laboratory, California Institute of Technology, under a contract with NASA.
References
Barr, A. C., V. Dobos, and L. L. Kiss (2018), Interior structures and tidal heating in the TRAPPIST-1 planets, Astron. Astrophys., 613, A37, https://doi.org/10.1051/0004-6361/201731992.
de Kleer, K., et al. (2019), Tidal heating: Lessons from Io and the Jovian system, final report, Keck Inst. for Space Studies, Pasadena, Calif., kiss.caltech.edu/final_reports/Tidal_Heating_final_report.pdf.
Fuller, J., J. Luan, and E. Quataert (2016), Resonance locking as the source of rapid tidal migration in the Jupiter and Saturn moon systems, Mon. Not. R. Astron. Soc., 458(4), 3,867–3,879, https://doi.org/10.1093/mnras/stw609.
Khurana, K. K., et al. (2011), Evidence of a global magma ocean in Io’s interior, Science, 332(6034), 1,186–1,189, https://doi.org/10.1126/science.1201425.
McEwen, A. S., et al. (2019), The Io Volcano Observer (IVO): Follow the heat!, Lunar Planet. Sci. Conf., 50, Abstract 1316, http://archive.space.unibe.ch/staff/wurz/McEwen_LPSC_1316.pdf.
Peale, S. J., P. Cassen, and R. T. Reynolds (1979), Melting of Io by tidal dissipation, Science, 203(4383), 892–894, https://doi.org/10.1126/science.203.4383.892.
Author Information
Alfred McEwen ([email protected]), University of Arizona, Tucson; Katherine de Kleer, California Institute of Technology, Pasadena; and Ryan Park, Jet Propulsion Laboratory, California Institute of Technology, Pasadena
Citation:
McEwen, A.,de Kleer, K., and Park, R. (2019), Does Io have a magma ocean?, Eos, 100, https://doi.org/10.1029/2019EO135617. Published on 18 October 2019.
Text © 2019. The authors. CC BY-NC-ND 3.0
Except where otherwise noted, images are subject to copyright. Any reuse without express permission from the copyright owner is prohibited.