As the world seeks strategies to reduce emissions of carbon dioxide (CO2) into the atmosphere, bioenergy is one promising substitute for fossil fuels [Somerville et al., 2010]. Currently, the United States uses the starch component from roughly 40% of its corn harvest to produce ethanol for the transportation sector (see the National Agricultural Statistics Service website).
Currently, the United States uses the starch component from roughly 40% of its corn harvest to produce ethanol for the transportation sector.
Cornstarch production is technologically simple—a so-called first-generation bioenergy technology. However, growing corn requires a lot of fertilizer and field preparation that ultimately depend on fossil fuels, tempering the net carbon savings.
Because of this, researchers have focused on developing fuel production using more advanced methods and second-generation bioenergy crops. These methods make liquid fuel, primarily ethanol, from lignocellulose, which composes the structural elements of plants, leaves, stalks, and stems. Because many of these crops are perennials (they grow back year after year), they often require less fertilizer and tillage, avoiding many of the negatives associated with corn and other annual crops that need intensive management.
A challenge with second-generation energy crops, however, is that they yield much less energy—lignocellulose produces less than one third of the energy per unit mass compared to fossil fuels. Because of this low energy density, the United States would need to invest a considerable amount of land to meet a significant part of national demand, a land area almost the size of Wisconsin to meet the Renewable Fuel Standard mandate for 32 billion gallons of biofuel [Hudiburg et al., 2015].
Planting second-generation grass-based biofuel crops on marginal or degraded land can reduce our carbon footprint and provide other beneficial ecosystem services.
The conversion of current land uses and management practices to the cultivation of bioenergy crops directly affects the climate system and is a fundamental process underlying the ecological sustainability of bioenergy production, as well as the ability of bioenergy crops to mitigate climate change. Where conversion of native prairie to corn negatively affects climate by releasing CO2 and other greenhouse gases to the atmosphere, planting second-generation grass-based biofuel crops on marginal or degraded land can reduce our carbon footprint and provide other beneficial ecosystem services.
Changing the Landscape Changes Our Climate
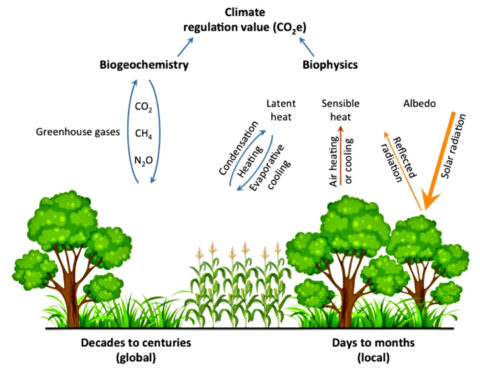
Public discussion of climate change often focuses on the atmosphere. As greenhouse gases (GHGs) like carbon dioxide, nitrous oxide, and methane accumulate in the atmosphere, they warm the planet by absorbing infrared radiation. But that’s not the whole picture: those greenhouse gases are also constantly cycling between the atmosphere and the land (Figure 1). Therefore, changes in land use, vegetation, and how we manage it affect climate by altering that exchange.
The metabolisms of plants and soil microbes help to regulate the exchange of GHGs with the atmosphere, as these molecules or their precursors are stored in biomass and soil. Clearing a native forest, for example, releases large quantities of carbon stored in biomass and soil to the atmosphere (“storage”; Figure 2). Indeed, creating and managing farmland contribute more than 14% of the world’s GHG emissions (U.S. Environmental Protection Agency, global greenhouse gas emissions data, 2015).
![Fig. 2. (a) Biogeochemical climate services reflect the greenhouse gases that would be released from land clearing and the change in ongoing exchange with the atmosphere. (b) Similarly, land clearing affects biophysical climate services, albedo, related to net radiation (Rnet) and latent heat flux from evaporation (LE), related to changes in evapotranspiration. Positive values represent net cooling effect on the atmosphere. (c) The sum of greenhouse gases and biophysical factors is the climate regulating value (CRV) of an ecosystem. Values are normalized to the warming potential of carbon dioxide (CO2) and are expressed relative to bare ground over a 50-year time frame. Replacing an ecosystem with a high CRV with that having a low value would have a net warming effect on the atmosphere and vice versa. Reproduced from Anderson-Teixiera et al. [2012].](https://eos.org/wp-content/uploads/2015/12/15-0377_DeLucia_4937_F02_WEB.jpg)
Terrestrial ecosystems also affect climate on local scales by regulating the exchange of energy between the land and atmosphere (Figure 1) [Davin et al., 2014; Zhao and Jackson, 2014]. Land covered with vegetation generally absorbs more sunlight than bare soil, contributing to local warming. Working against this, when water evaporates and passes into the atmosphere from soil and vegetation (known collectively as evapotranspiration), it carries heat away from the land, causing local cooling. Thus, forest clearing would reduce the cooling effect of evapotranspiration but would increase the reflectivity of the surface (or its albedo), allowing it to reflect more radiation.
However, global effects are complicated because evapotranspiration means more moisture in the atmosphere, which can increase cloud cover, affecting the global radiation balance. Also, when the moisture condenses into clouds elsewhere, it releases its latent heat, which can cancel the local cooling effect [Pielke et al., 2002; Snyder et al., 2004]. Unlike GHGs that have a locally weak but globally strong effect on the atmosphere, albedo and evapotranspiration affect climate locally [Bright, 2015].
By normalizing the warming (or cooling) potential of nitrous oxide (N2O), methane, albedo, and evapotranspiration to the warming potential of CO2, the contribution of different ecosystems to climate regulation can be expressed as a single metric: the climate regulating value (CRV) [Anderson-Teixeira et al., 2012]; this value provides an integrated index of the direct effects of land clearing on the surface energy budget, where the greater the CRV is, the greater the cooling effect is (Figure 2).
Long-Term Strategic Planting
In native forests and other ecosystems with large carbon stocks, biogeochemical processes—those processes that store and exchange GHGs with the atmosphere—can play a larger role in regulating the climate than biophysical processes such as changes in evapotranspiration and albedo (Figure 2). The opposite can be true for perennial grasses that don’t store much biomass but have high evapotranspiration and albedo.
Therefore, displacing native forests, particularly tropical forests, with annual or perennial crops for energy production will, in most cases, have a net warming effect on the atmosphere, releasing large quantities of carbon stored in biomass and soil (Figure 2). But replacing annual crops or placing high-yield bioenergy crops on marginal land (that is, land that cannot produce high-value crops) has a very different effect.
Replacing annual crops with perennial grasses would pull carbon out of the atmosphere and return it to the ground.
Replacing annual crops with perennial grasses such as miscanthus and switchgrass would pull carbon out of the atmosphere and return it to the ground (Figure 2). These crops allocate a large fraction of their biomass below ground in their root systems, and they can rapidly build up carbon stores in soil, reversing losses associated with frequent tillage, particularly on degraded or heavily tilled soils [Anderson-Teixeira et al., 2009, 2013; Powlson et al., 2011].
In terms of climate effects, this is doubly helpful. In addition to displacing fossil fuels by providing a renewable biofuel, replacing low-CRV annual crops with high-CRV perennial grasses would have a net cooling effect on the atmosphere because of the changes in biogeochemical and biophysical properties. In particular, the increased albedo from perennial grass and the heat carried away by evapotranspiration can amount to a considerable cooling effect compared to annual row crops [Georgescu et al., 2011].
The U.S. Midwest: From Carbon Source to Sink
Of the approximately 40 million hectares in the United States that are planted with corn, mostly in the Midwest, only 8% of them directly feeds humans; most of the rest (73%) is for feeding livestock and producing ethanol (National Agricultural Statistics Service website, 2015). Displacing corn currently grown for ethanol with high-yielding perennial grasses would have enormous environmental benefits, without displacing land used for food production. Davis et al. [2012] predict that replacing ethanol-bound corn with perennial grasses would reduce emissions of GHGs to the atmosphere while increasing soil carbon. The emissions of N2O in particular would be reduced because perennial grasses require so much less nitrogen than corn [Smith et al., 2013]. Over the entire region, this transition would convert soils in the Midwest from a net source to a net sink for GHGs while simultaneously increasing fuel production and reducing the contamination of groundwater by fertilizer-derived nitrate.
Prime corn land is expensive. Restricting most bioenergy grasses to more affordable marginal land would also drive a reduction in U.S. GHG emissions, albeit a smaller one than replacing corn ethanol, and would still meet the Renewal Fuel Standard’s mandate for 32 billion gallons of renewable biofuel, with negligible effects on food crop production [Hudiburg et al., 2015].
The biophysical processes will also help to regulate climate. Evapotranspiration would increase slightly—less than 10% [VanLoocke et al., 2010]—but combined with an increased albedo, that’s enough to provide an additional local cooling effect.
The Promise and Challenges of a Bioenergy Landscape
In addition to displacing CO2 emitted from fossil fuels, the expansion of perennial bioenergy crops in the U.S. Midwest will likely have positive effects on the climate system. This, however, is not necessarily the case elsewhere. In much of the United States west of the 100th meridian (a line that roughly bisects the Dakotas and Texas), the ability of the atmosphere to remove water (potential evapotranspiration) exceeds precipitation. There, the irrigation necessary for energy crops would pose severe environmental challenges.
In Southeast Asia, displacing native forest with palm oil plantations, in part for biodiesel, has increased the amount of atmospheric GHGs and hurt biodiversity [Danielsen et al., 2009]. Furthermore, by increasing grain prices, displacing food crops with bioenergy crops may encourage deforestation in the tropics for expanding agriculture [Searchinger et al., 2008].
We must take care to avoid the unintended negative consequences of expanding lignocellulosic bioenergy production.
We must take care to avoid these unintended negative consequences of expanding lignocellulosic bioenergy production. But with appropriate financial incentives [Dwivedi et al., 2015], there are many strategies to use land sustainably to contribute to the U.S. demand for transportation fuel: the use of marginal or underproductive lands [Gelfand et al., 2013] or replacing intensively managed corn for ethanol with high-yielding, low-input perennials. When annual crops that require intensive management are replaced with high-yielding perennial plants, bioenergy crops can simultaneously reduce the emission of GHGs to the atmosphere and improve the health of agricultural landscapes [Werling et al., 2014].
References
Anderson-Teixeira, K. J., S. C. Davis, M. D. Masters, and E. H. DeLucia (2009), Changes in soil organic carbon under biofuel crops, Global Change Biol. Bioenergy, 1, 75–96.
Anderson-Teixeira, K. J., P. K. Snyder, T. E. Twine, C. V. Cuadra, M. H. Costa, and E. H. DeLucia (2012), Climate-regulation services of natural and agricultural ecoregions of the Americas, Nat. Clim. Change, 2, 177–181, doi:10.1038/NCLIMATE1346.
Anderson-Teixeira, K. J., M. M. Masters, C. J. Black, M. Zeri, M. Z. Hussain, C. J. Bernacchi, and E. H. DeLucia (2013), Altered belowground carbon cycling following land-use change to perennial bioenergy crops, Ecosystems, 16, 508–520.
Bright, R. M. (2015), Metrics for biogeophysical climate forcings from land use and land cover changes and their inclusion in life cycle assessment: A critical review, Environ. Sci. Technol., 49, 3291–3303.
Danielsen, F., H. Beukema, N. D. Burgess, F. Parish, C. A. Brühl, P. F. Donald, D. Murdiyarso, B. Phalan, L. Reijinders, M. Struebig, and E. B. Fitzherbert (2009), Biofuel plantations on forested lands: Double jeopardy for biodiversity and climate, Conserv. Biol., 23, 348–358.
Davin, E. L., S. I. Seneviratne, P. Ciais, A. Olioso, and T. Wang (2014), Preferential cooling of hot extremes from cropland albedo management, Proc. Natl. Acad. Sci. U. S. A., 111, 9757–9761.
Davis, S. C., W. J. Parton, S. J. Del Grosso, C. Keogh, E. Marx, and E. H. DeLucia (2012), Impact of second-generation biofuel agriculture on greenhouse-gas emissions in the corn-growing regions of the US, Front. Ecol. Environ., 10, 69–74.
Dwivedi, P., et al. (2015), Cost of abating greenhouse gas emissions with cellulosic ethanol, Environ. Sci. Technol., 49, 2512–2522.
Gelfand, I., R. Sahajpal, X. Zhang, R. C. Izaurralde, K. L. Gross, and G. P. Robertson (2013), Sustainable bioenergy production from marginal lands in the US Midwest, Nature, 493, 514–520.
Georgescu, M., D. B. Lobell, and C. B. Field (2011), Direct climate effects of perennial bioenergy crops in the United States, Proc. Natl. Acad. Sci. U. S. A., 108, 4307–4312.
Hudiburg, T. W., W. W. Wang, M. Khanna, S. P. Long, P. Dwivedi, W. J. Parton, M. Hartmann, and E. H. DeLucia (2015), Impacts of a 32-billion-gallon bioenergy landscape on land and fossil fuel use in the US, Nat. Energy, in press.
Jobbágy, E. G., and R. B. Jackson (2000), The vertical distribution of soil organic carbon and its relation to climate and vegetation, Ecol. Appl., 10, 423–436.
Pielke, R. A., et al. (2002), The influence of land-use change and landscape dynamics on the climate system: Relevance to climate-change policy beyond the radiative effect of greenhouse gases, in Capturing Carbon and Conserving Biodiversity: A Market Approach, edited by I. R. Swingland, pp. 157–172, Earthscan Publ., London.
Powlson, D. S., A. P. Whitmore, and W. W. T. Goulding (2011), Soil carbon sequestration to mitigate climate change: A critical re-examination to identify true and false, Eur. J. Soil Sci., 62, 42–55.
Searchinger, T., R. Helmlich, R. A. Houghton, F. Dong, A. Elobeld, J. Fabiosa, S. Tokgoz, D. Hayes, and T.-H. Yu (2008), The use of U.S. croplands for biofuels increases greenhouse gases through emissions from land-use change, Science, 319, 1238–1240.
Smith, C. M., et al. (2013), Reduced nitrogen losses after conversion of row crop agriculture to perennial bioenergy crops, J. Environ. Qual., 42, 219–228.
Snyder, P. K., C. Delire, and J. A. Foley (2004), Evaluating the influence of different vegetation biomes on the global climate, Clim. Dyn., 23, 279–302.
Somerville, C., H. Youngs, C. Taylor, S. C. Davis, and S. P. Long (2010), Feedstocks for lignocellulosic biofuels, Science, 329, 790–792.
VanLoocke, A., C. J. Bernacchi, and T. E. Twine (2010), The impacts of Miscanthus x giganteus production on the Midwestern US hydrologic cycle, Global Change Biol. Bioenergy, 2, 180–191.
Werling, B. P., et al. (2014), Perennial grasslands enhance biodiversity and multiple ecosystem services in bioenergy landscapes, Proc. Natl. Acad. Sci. U. S. A., 111, 1652–1657.
Zhao, K., and R. B. Jackson (2014), Biophysical forcings of land-use change from potential forestry activities in North America, Ecol. Monogr., 84, 329–353.
Author Information
Evan H. DeLucia, Department of Plant Biology, Institute for Sustainability, Energy, and Environment, Energy Biosciences Institute and Carl R. Woese Institute for Genomic Biology, University of Illinois at Urbana–Champaign; email: [email protected]
Citation: DeLucia, E. H. (2015), How biofuels can cool our climate and strengthen our ecosystems, Eos, 96, doi:10.1029/2015EO041583. Published on 22 December 2015.
Correction, 22 December 2015: An earlier version of this article incorrectly listed Carl R. Woes as a coauthor. Evan H. DeLucia is affiliated with the Carl R. Woese Institute for Genomic Biology, University of Illinois at Urbana–Champaign.
Correction, 6 January 2016: The caption of the main image accompanying this feature article has been updated to reflect that miscanthus and switchgrass are perennial rather than annual grasses.
Text © 2015. The authors. CC BY-NC 3.0
Except where otherwise noted, images are subject to copyright. Any reuse without express permission from the copyright owner is prohibited.