From March to May 1980, magma rose high into Mount St. Helens (MSH), swelling and—as it turned out—destabilizing its north flank. Scientists knew the volcano had been highly active at times over the past 40,000 years, but the mountain, located amid the Cascade Range in southwestern Washington, had been mostly quiet since the mid-19th century. The collapse of the north flank on 18 May shattered that quiet, triggering a cascade of events that left resounding impressions not only on those who witnessed and studied them but also on the surrounding landscape [Lipman and Mullineaux, 1981; Waitt, 2015].
40 Years of Science at Mount St. Helens
The eruption of MSH also provided unparalleled opportunities for advancing several disciplines [e.g., Shore et al., 1986; Newhall, 2000; Franklin and MacMahon, 2000]. Among these was an intensification of research investigating biophysical impacts of eruptions and subsequent responses [e.g., Dale et al., 2005; Pierson and Major, 2014; Crisafulli and Dale, 2018]. Long-term research on the biophysical responses at MSH has provided important new insights, challenged long-standing ideas, and provided many societal benefits.
The fortieth anniversary of the eruption this year offers a timely opportunity to reflect on these insights and influences. This long-term vantage is important because sustained, place-based studies following landscape disturbances are rare; because the MSH eruption spurred the greatest depth and breadth of multidisciplinary studies of biophysical responses to landscape disturbance; and because these responses created some of the most significant societal challenges to emerge after the eruption. We summarize key biophysical disturbances and responses, highlight salient insights, and suggest actions that can extend the usefulness of these insights to volcanically vulnerable communities worldwide.
Smothered, Battered, and Singed
The flank collapse at MSH on the morning of 18 May initiated several catastrophic events over the next several minutes (Figure 1). The resulting colossal landslide smothered 60 square kilometers of river valley to a mean depth of 45 meters, obstructed tributaries to the North Fork Toutle River, or NFTR (consequently impounding two permanent and several ephemeral lakes), and blocked the outlet of Spirit Lake at the foot of the volcano. An associated energy blast from the eruption unleashed a scorching cloud of rocky debris known as a pyroclastic density current (hereinafter called the blast PDC) that swept over 600 square kilometers of rugged mountain terrain, removing, toppling, and singeing tracts of forest. Parts of that cloud also sped down the volcano’s snowclad east and west flanks, triggering meltwater flash floods that swept up sediment to become large, swift volcanic mudflows (lahars) that traveled many tens of kilometers downstream.
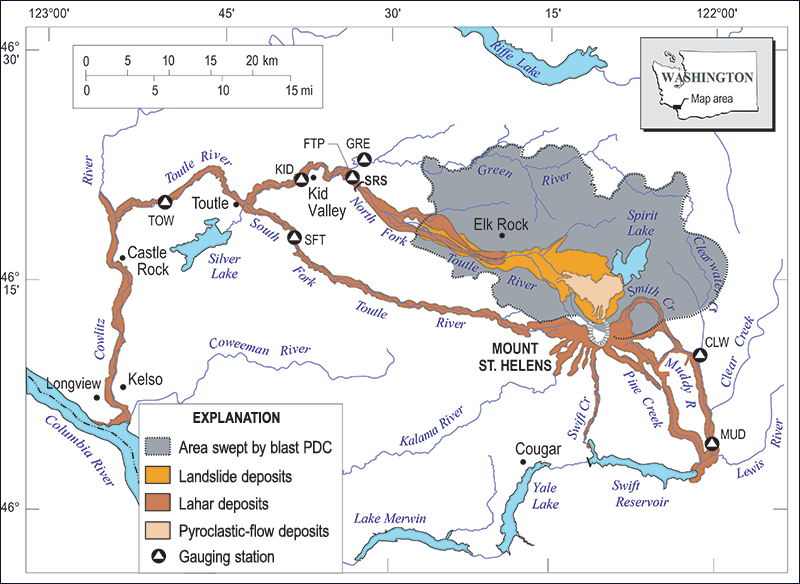
A vigorous vertical eruption plume soon followed these events, raining shards of volcanic debris (tephra fall) downwind and producing pumice-rich pyroclastic flows that settled atop the landslide deposit. Hours after the landslide emplacement, parts of the newly deposited material liquefied, forming muddy slurries that coalesced into the massive NFTR lahar that reached distant communities and choked navigation on the Columbia River more than 100 kilometers downstream from the volcano.
Erosion, burial, heat, blunt force, and abrasion produced a mosaic of landscape disturbances that extended tens of kilometers from the volcano.
These eruption processes battered forest, meadow, riparian, riverine, lake, and lakeshore environments. Erosion, burial, heat, blunt force, and abrasion produced a mosaic of landscape disturbances that extended tens of kilometers from the volcano. The magnitude of disturbances ranged widely, from the near-total removal of all vestiges of plants and animals in areas nearest the volcano to terrain dusted with only a thin layer of tephra. This patchwork of disturbances created an exceptional natural experiment for assessing physical and biological responses to spatially variable impacts.
The Backdrop for Biophysical Research
Biophysical research at MSH following the eruption was influenced by prevailing ideas in 1980 of how landscapes and ecosystems change in response to large disturbances, by the state of the new landscape, and by management of public lands at the volcano. In 1980, U.S. scientists had little direct experience with explosive volcanism or biophysical responses to eruptions. Lahars were a known volcanic process, but scientists poorly understood how they evolved downstream and how landscapes responded to other hydrogeomorphic impacts, such as riverine responses to vast injections of sediment or modifications to basin hydrology caused by expansive changes in forest cover, tephra deposition, and channel disruption. Meanwhile, conventional ecological wisdom held that most organisms would likely perish where intense volcanic forces affected the landscape. It was also thought that recovery of decimated areas would be regulated by their distance from neighboring, unaffected areas, from which hardy pioneering species could migrate and reestablish conditions favorable for later successional species [Franklin and MacMahon, 2000].
The 1980 eruption upended the normal hydrogeomorphic functioning of much of the landscape. Loss of hillside vegetation and deposition of impermeable silty tephra promoted rapid surface water and sediment runoff; immense amounts of material introduced to stream channels disrupted major river corridors; landslide debris tenuously dammed existing and developing lakes; and lahar deposits compromised navigation and flood protection capacity in distant communities by raising channel beds. These conditions created intense concerns over public safety related to flood and sediment hazards and afforded needs and opportunities for subsequent studies.
Prior to the Mount St. Helens eruption, ecologists had commonly studied organism responses years to centuries after an eruption.
Prior to the MSH eruption, ecologists had commonly studied organism responses years to centuries after an eruption and typically had studied single groups of organisms affected by single volcanic processes or deposits. After the eruption, the mosaic of different volcanic deposits and widely varying impacts to numerous organisms across multiple environments afforded ecologists opportunities to make significant strides in understanding linkages among disturbance, survival, and succession.
Research efforts at MSH have influenced, and have been affected by, political and institutional processes that determined human engagement with this new landscape. In 1982, Congress created the 445-square-kilometer Mount St. Helens National Volcanic Monument (NVM), identifying science, education, and recreation as the primary objectives of land management. The U.S. Forest Service was charged with “allowing geologic forces and ecological succession to continue substantially unimpeded,” except as necessary to ensure public safety. Meeting this charge has entailed striking a delicate balance among competing interests, including public desire for full access, minimization of access to certain areas to protect ecological research values, and the NVM’s responsibility to manage geologic hazards to ensure protection of public safety and the environment. It’s a challenge complicated by changing landscape conditions, evolving social values, and emerging scientific knowledge.
A Landscape Reshaped
Geomorphic responses to the landscape disturbances far outweighed hydrological responses. The nature, magnitude, and duration of geomorphic responses around MSH varied substantially and reflect the types and severities of different eruption processes. Stormflows from basins that were heavily affected by the eruption, however, increased by a few percent to a few tens of percent compared with pre-eruption flows for roughly 5–10 years [Major and Mark, 2006].
The most substantial geomorphic response occurred in the NFTR basin, which was affected by all the eruption processes; this response continues today because the basin’s upper valley was smothered in thick deposits. In the NFTR basin, water runoff, small lake breakouts, and water pumped from Spirit Lake (to lower the lake level from 1982 to 1985 until an outlet was constructed) efficiently carved the landslide and pyroclastic flow deposits, leading to exceptional erosion of new channels (tens of meters of incision and hundreds of meters of widening) and driving extreme levels of sediment delivery (Figure 2). Although that extraordinary sediment yield declined rapidly as channels widened and beds coarsened, it remains elevated decades later because of ongoing, low-magnitude lateral erosion of the deep channels [Major et al., 2018].
In comparison, in basins affected solely by the blast PDC and tephra fall, stream channel responses were minor and hillside erosion decreased swiftly owing to mechanically driven (versus vegetation-driven) increases in infiltration and the complex topography created by downed trees [Collins and Dunne, 2019]. In those basins, such as the Green River and Clearwater Creek basins, elevated sediment delivery was brief and returned to background levels within about 5 years (Figure 2). After 40 years, more than 80% of all MSH eruption-deposited sediment remains in place—and much of that might remain permanently—but problematic sediment delivery from the NFTR basin will likely persist for decades to come.
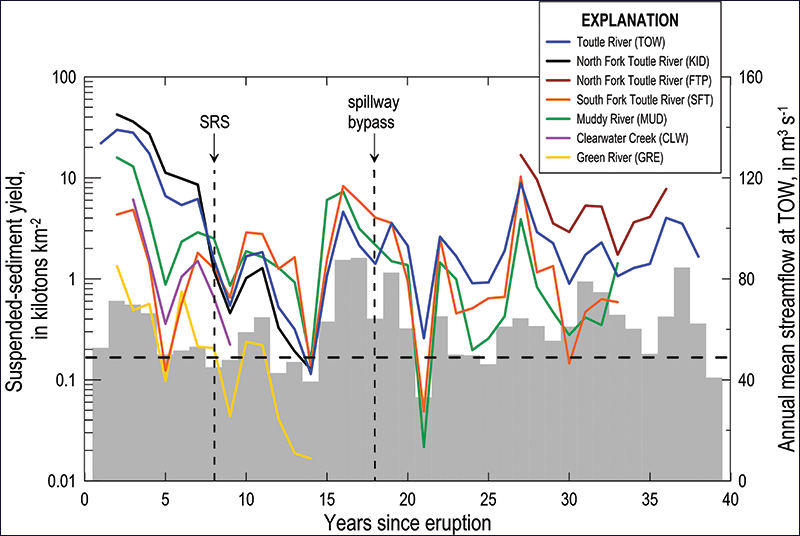
Hydrogeomorphic responses to volcanic disturbances can result in socioeconomic consequences more damaging than the direct impacts of eruptions themselves [Pierson and Major, 2014]. The great flush of sediment into waterways around MSH, to date more than 400 million tons, has compounded eruption impacts, greatly prolonging navigation problems and flood hazards to vulnerable communities, for example. This forced the U.S. Army Corps of Engineers (ACOE) to conduct extensive, but unsustainable, channel dredging [Willingham, 2005]. In 1989, ACOE completed a 56-meter-tall, 800-meter-long sediment-retention dam to minimize sediment reaching the Cowlitz and Columbia Rivers (Figure 1). But persistent erosion and sediment delivery have proved to be a formidable foe; by 1998, sediment behind the dam reached the spillway level, prompting further efforts to curtail sediment delivery downstream [Sclafani et al., 2018]. To date, ACOE has spent more than $435 million mitigating eruption and post-eruption impacts on the Cowlitz and elsewhere, and no immediate end is in sight.
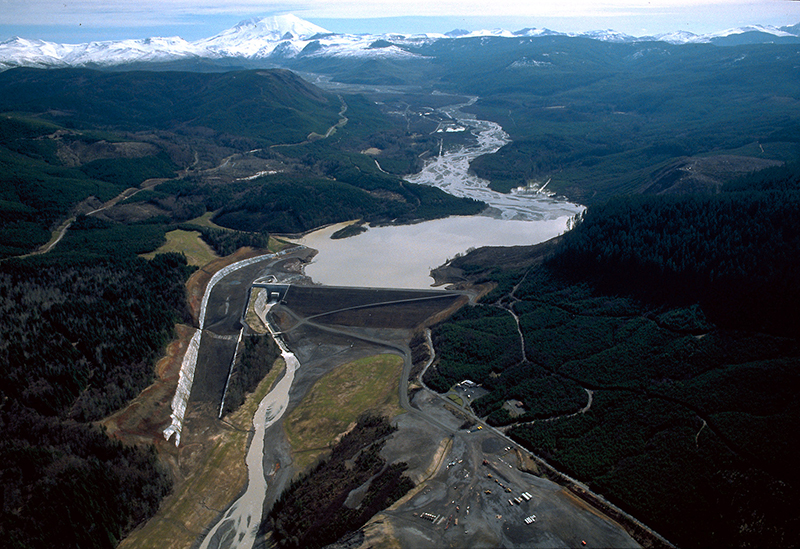
Insights from hydrogeomorphic studies at MSH have had wide influence. They have sharpened global understanding of post-eruption landscape functioning by elucidating the nature and pace of landscape responses, and they have informed flood management planning and policies locally by quantifying magnitudes and durations of sediment delivery and by identifying sources of sediment erosion and sinks of deposition. These studies have also helped hone interpretations of hydrogeomorphic responses to other types of disturbances, such as wildfires (which in some ways mimic those following tephra falls) and abrupt injections of channel sediment following large-dam removals and mining.
Legacies Live On
After the eruption, ecologists quickly discovered that biological legacies (surviving plants and animals) from pre-eruption ecosystems persisted and were widely distributed; even some of the most heavily affected landscapes were not as sterile as initially assumed. The seasonal timing of the eruption and its time of day played important roles in determining which plants and animals lived or died; many organisms in subalpine lakes and on hillsides, protected beneath snow or ice, were spared the brunt of eruptive forces, for example, and nocturnal animals were in their dens. Those protections resulted in numerous patches of surviving organisms embedded within a vast expanse of disturbed land (Figure 3).
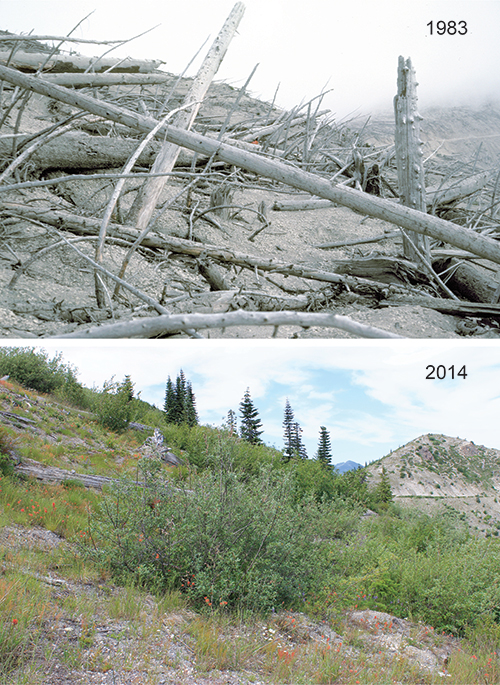
Researchers found that the types, amounts, and distributions of biological legacies were the most important factors affecting rates of ecological recovery [Dale et al., 2005; Crisafulli and Dale, 2018]. But ecological recovery also involved colonizing organisms. Forests and aquatic systems surrounding the disturbed landscape were largely intact and supported plants and animals that served as source populations for post-eruption recovery [Crisafulli and Dale, 2018]. These populations were particularly important for the recovery of species that experienced complete mortality in the blast PDC zone, such as many large mammals and birds.
Certain species, called keystone species, were exceptionally important in the recovery process. For example, lupines and alders flourished in the nutrient-impoverished volcanic substrates because of their ability to partner with root-borne, nitrogen-fixing bacteria. These pioneer species facilitated colonization of many other plants and animals by ameliorating inhospitable nutrient conditions and initiating soil development. Other species, such as the northern pocket gopher, facilitated recovery by mixing inert tephra with buried nutrient-rich soil, thus improving conditions for plant growth. American beavers modified streamflow and plant communities through herbivory and dam building, which created habitats with high biodiversity.
Abiotic factors were also important to ecological recovery. The unconsolidated texture of eruption deposits allowed animals to excavate burrows. Surviving plants penetrated thin (50 centimeters or less) deposits by sprouting new shoots upward, and roots of seedlings penetrated downward into nutrient-rich pre-eruption soils. The mild, wet maritime climate at MSH further facilitated ecological response.
The importance of biological legacies in promoting recovery emerged as an epiphany from long-term ecological research at Mount St. Helens.
Lessons from long-term ecological research at MSH have advanced fundamental understanding of how individual species and biological communities respond to large, intense disturbances. Locally, this work has assisted management of NVM lands. More broadly, it has allowed ecologists to address a fundamental question in ecology: How do biological communities arise from a seemingly barren slate? The importance of biological legacies in promoting recovery emerged as an epiphany, one that has informed research at other volcanoes [Crisafulli et al., 2015] and at sites experiencing intense wildfires and windstorms. The work has also prompted fresh perspectives on management of forest landscapes in the United States and abroad, such as using variable-retention harvesting, rather than clear-cutting, to preserve forest structures and processes [Franklin and Donato, 2020].
Feedbacks Between Life and Land
Geomorphic and ecologic responses at MSH have evolved jointly and interacted in important ways. For example, on hillsides affected by the blast PDC, concentrated surface runoff eroded tephra, which exposed surviving seeds and rootstocks in pre-eruption soil and allowed them to flourish (Figure 4). Trees and shrubs are now widespread in some basins and have established enough cover, root mass, and strength to help anchor hillside tephra deposits, which minimizes additional erosion by runoff and landslides.
A similar example is seen along river corridors, where a certain degree of geomorphic stability has been needed before riparian vegetation could effectively be established. As that riparian vegetation has matured and gained stronger footholds, it has increased geomorphic stability. Furthermore, the combination of increased geomorphic stability and expanding riparian vegetation has helped establish animal communities. Areas where channel banks and floodplains remain unstable support little vegetation and few animals.
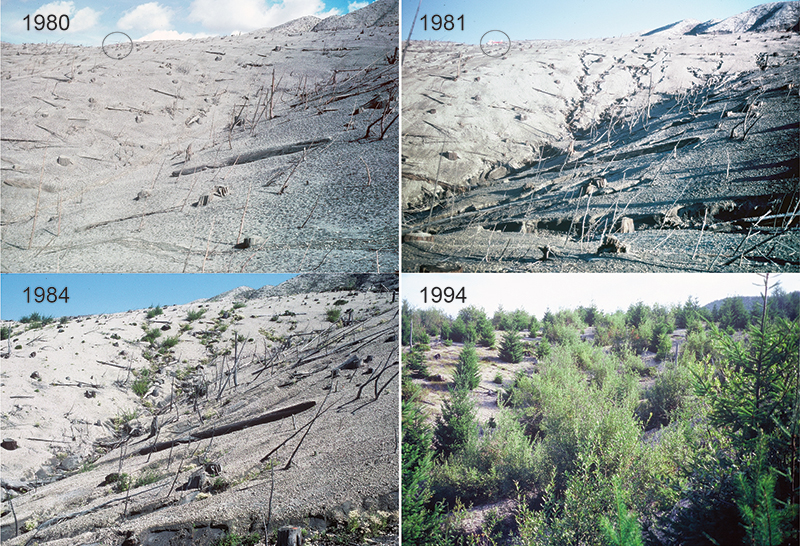
A Call to Action
Results from 4 decades of hydrogeomorphic and ecological research at MSH affirm the value of long-term monitoring and research at disturbed landscapes, as well as of the establishment of collaborative research communities. This multidisciplinary research continues to inform not only basic science but also societally important endeavors to understand and manage disturbed landscapes. These endeavors include efforts to manage flooding and sediment delivery in waterways affected by the eruption—especially as mitigation measures have failed or become less effective with age—and to manage native and invasive species on NVM lands. The research has also provided important, factual information about the eruption that is shared with the public at local visitor centers and through global media.
As populations living near volcanoes increase, more can be done to impart lessons learned from biophysical research at Mount St. Helens.
As populations living near volcanoes increase, more can be done to impart lessons learned from biophysical research at MSH. We propose five steps for the volcano science community to take that would extend the reach of these lessons:
- Improve public awareness in communities vulnerable to volcanic hazards of the biophysical responses to eruptions. Scientists can fold discussions of these responses into other volcano hazard awareness efforts, such as workshops and public presentations.
- Include instruction about biophysical impacts and responses to eruptions in academic courses focused on natural hazards to pass on the knowledge gained to future generations of students and scientists.
- Include post-eruption sedimentation hazards in volcano hazards assessments to more accurately portray the full range and duration of hazards associated with volcanism.
- Develop an international volcano ecology and hydrology network—either informally or formally through scientific societies—to connect ecologists, hydrologists, and geomorphologists; facilitate information sharing; and identify key issues that arise in post-eruption landscapes.
- Foster collaborations among hydrologists, geomorphologists, and ecologists who can consult with local volcano observatories and provide training for local science agencies, civil authorities, and emergency managers. Training could address what to anticipate in the immediate and long-term aftermath of future eruptions, appropriate hazard assessment methods, possible options and approaches to mitigating posteruptive hydrogeomorphic hazards, and advice for restoring ecosystems to desired states. The U.S. Geological Survey’s Volcano Disaster Assistance Program [Lowenstern and Ramsey, 2017], which is funded by the U.S. Agency for International Development, is one example of such a team; international scientific societies could facilitate others.
Following these steps can further advance the rich scientific understanding of the myriad and dynamic processes that occur in post-eruption landscapes, which studies at Mount St. Helens since 1980 have sharpened, and could help reveal how this understanding can best be applied for the benefit of public safety and the environment.
References
Collins, B. D., and T. Dunne (2019), Thirty years of tephra erosion following the 1980 eruption of Mount St. Helens, Earth Surf. Processes Landforms, 44(14), 2,780–2,793, https://doi.org/10.1002/esp.4707.
Crisafulli, C. M., and V. H. Dale (Eds.) (2018), Ecological Responses at Mount St. Helens: Revisited 35 Years After the 1980 Eruption, 336 pp., Springer, New York, https://doi.org/10.1007/978-1-4939-7451-1.
Crisafulli, C. M., et al. (2015), Volcano ecology: Disturbance characteristics and assembly of biological communities, in The Encyclopedia of Volcanoes, 2nd ed., edited by H. Sigurdsson et al., chap. 73, pp. 1,265–1,284, Elsevier, Amsterdam, https://doi.org/10.1016/C2015-0-00175-7.
Dale, V. H., F. J. Swanson, and C. M. Crisafulli (Eds.) (2005), Ecological Responses to the 1980 Eruption of Mount St. Helens, 342 pp., Springer, New York, https://doi.org/10.1007/0-387-28150-9.
Franklin, J. F., and D. C. Donato (2020), Variable retention harvesting in the Douglas-fir region, Ecol. Processes, 9, 8, https://doi.org/10.1186/s13717-019-0205-5.
Franklin, J. F., and J. A. MacMahon (2000), Messages from a mountain, Science, 288(5469), 1,183–1,184, https://doi.org/10.1126/science.288.5469.1183.
Lipman, P. W., and D. R. Mullineaux (Eds.) (1981), The 1980 eruptions of Mount St. Helens, Washington, U.S. Geol. Surv. Prof. Pap., 1250, 844 pp., https://doi.org/10.3133/pp1250.
Lowenstern, J. B., and D. W. Ramsey (2017), The Volcano Disaster Assistance Program—Helping to save lives worldwide for more than 30 years, U.S. Geol. Surv. Fact Sheet, 2017-3071, 6 pp., https://doi.org/10.3133/fs20173071.
Major, J. J., and L. E. Mark (2006), Peak flow responses to landscape disturbances caused by the cataclysmic 1980 eruption of Mount St. Helens, Washington, Geol. Soc. Am. Bull., 118, 938-958, https://doi.org/10.1130/B25914.1.
Major, J. J., A. R. Mosbrucker, and K. R. Spicer (2018), Sediment erosion and delivery from Toutle River basin after the 1980 eruption of Mount St. Helens: A 30-year perspective, in Ecological Responses at Mount St. Helens: Revisited 35 Years After the 1980 Eruption, edited by C. M. Crisafulli and V. H. Dale, pp. 19–44, Springer, New York, https://doi.org/10.1007/978-1-4939-7451-1_2.
Newhall, C. G. (2000), Mount St. Helens, master teacher, Science, 288(5469), 1,181–1,183, https://doi.org/10.1126/science.288.5469.1181.
Pierson, T. C., and J. J. Major (2014), Hydrogeomorphic effects of explosive volcanic eruptions on drainage basins, Annu. Rev. Earth Planet. Sci., 42(1), 469–507, https://doi.org/10.1146/annurev-earth-060313-054913.
Sclafani, P., C. Nygaard, and C. Thorne (2018), Applying geomorphological principles and engineering science to develop a phased sediment management plan for Mount St. Helens, Washington, Earth Surf. Processes Landforms, 43(5), 1,088–1,104, https://doi.org/10.1002/esp.4277.
Shore, J. H., E. L. Tatum, and W. M. Vollmer (1986), Evaluation of mental effects of disaster, Mount St. Helens eruption, Am. J. Public Health, 76, 76–83, https://doi.org/10.2105/AJPH.76.Suppl.76.
Waitt, R. (2015), In the Path of Destruction: Eyewitness Chronicles of Mount St. Helens, 416 pp., Wash. State Univ. Press, Pullman.
Willingham, W. F. (2005), The Army Corps of Engineers’ short-term response to the eruption of Mount St. Helens, Ore. Hist. Q., 106(2), 174–203, https://www.jstor.org/stable/20615526.
Author Information
Jon J. Major ([email protected]), Cascades Volcano Observatory, U.S. Geological Survey, Vancouver, Wash.; Charles M. Crisafulli, Pacific Northwest Research Station, U.S. Forest Service, U.S. Department of Agriculture, Amboy, Wash.; and Frederick J. Swanson (Scientist Emeritus), Pacific Northwest Research Station, U.S. Forest Service, U.S. Department of Agriculture, Corvallis, Ore.
Citation:
Major, J. J.,Crisafulli, C. M., and Swanson, F. J. (2020), Lessons from a post-eruption landscape, Eos, 101, https://doi.org/10.1029/2020EO143198. Published on 24 April 2020.
Text not subject to copyright.
Except where otherwise noted, images are subject to copyright. Any reuse without express permission from the copyright owner is prohibited.
Text not subject to copyright.
Except where otherwise noted, images are subject to copyright. Any reuse without express permission from the copyright owner is prohibited.