Magnetic storms are potentially hazardous to the activities and technological infrastructure of modern civilization. This reality was dramatically demonstrated during the great magnetic storm of March 1989, when surface geoelectric fields, produced by the interaction of the time-varying geomagnetic field with the Earth’s electrically conducting interior, coupled onto the overlying Hydro-Québec electric power grid in Canada. Protective relays were tripped, the grid collapsed, and about 9 million people were temporarily left without electricity [Bolduc, 2002].
A magnetic storm that was, by some measures, the most intense ever recorded followed a solar flare observed by astronomers Richard Carrington and Richard Hodgson in September 1859. Should a storm of similar intensity occur today, technological systems around the world could be adversely affected. According to some scenarios, the future occurrence of a rare “perfect magnetic storm” might cause widespread failure of bulk electric power networks (see Figure 1), with deleterious impact on the American economy and security [e.g., Baker et al., 2008].
Public and private agencies have responded to these findings. Notably, in May 2013, the Department of Energy’s Federal Energy Regulatory Commission (FERC) directed, through Order 779, the North American Electric Reliability Corporation to develop reliability standards to address the potential impact of geomagnetic disturbances on the operation of the bulk power system. Concerns in the private sector have motivated reinsurance companies to commission related assessments of risk.
As part of an interagency project coordinated under the auspices of the U.S. National Space Weather Program (NSWP), the U.S. Geological Survey (USGS), the National Oceanic and Atmospheric Administration (NOAA), and NASA are working together to improve regional assessments and real-time operational estimation of natural induction hazards that can be realized at the Earth’s surface during magnetic storms.
The Natural Hazard
The geophysical quantity that directly interferes with the operation of electric power grids is the geoelectric field. It is generated in the Earth’s interior through geomagnetic induction driven by magnetic activity originating overhead in the magnetosphere and ionosphere.
Electrical conductivity in the Earth’s interior ranges from about 10-4 siemens per meter (S/m) in some parts of the upper mantle to 3 S/m in the ocean. Generally speaking, electric power grids are susceptible to interference from naturally induced geoelectric fields that vary with periods from about 10 to 1000 seconds. Geomagnetic and geoelectric field variation over such periods plumbs the Earth’s interior across diffusive depth and length scales of between about 2 and 3000 kilometers, but localized conductivity anomalies can reduce the upper length scale to about 50 kilometers.
As a subject of natural science, estimating the geoelectric field as a function of geographic location is distinct from the engineering subject of electric currents that flow in power grids in response to geoelectric fields [e.g., Kappenman, 2001; Pirjola, 2002]. Mapping the time-dependent geoelectric field is needed to evaluate the design, emplacement, and ever-evolving operation of electric power grids [e.g., Viljanen et al., 2012]. Here we focus on the present-day challenges and opportunities for studying and quantifying hazardous geoelectric induction.
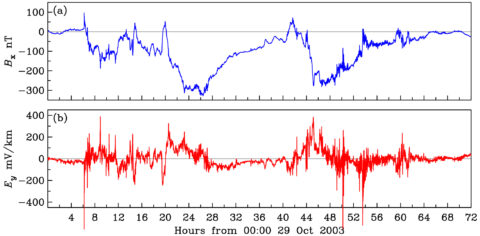
Geomagnetic Monitoring
Magnetometers around the world, such as those that are part of the International Real-time Magnetic Observatory Network (INTERMAGNET), record the temporal evolution of the geomagnetic field [Love and Chulliat, 2013]; an example time series is shown in Figure 2(a). As part of NSWP, the USGS magnetic observatory network produces high-quality magnetometer data for real-time nowcasting of magnetic storm conditions [Love and Finn, 2011]. Similarly integrated geomagnetic monitoring projects are supported in other countries, such as in Canada, the United Kingdom, and Japan.
Most national magnetic observatory networks are relatively sparse in geographic distribution. For example, the USGS operates only six stations in the lower continental United States, which is sufficient to resolve geomagnetic activity on a continental scale and to assess the general dynamic state of the magnetosphere-ionosphere system. More detailed analyses can exploit data from variometer magnetometer networks that are operated for specialized space weather projects [e.g., Viljanen et al., 2004; Yumoto et al., 2012].
Geoelectric Measurements
Direct measurement of the geoelectric field is conceptually simple: The voltage between a pair of electrodes, planted straight into the ground, is measured as a function of time. For magnetotelluric studies, geoelectric measurements are conventionally made in conjunction with magnetic variometer measurements, with sensors deployed over specific geographic regions on a temporary basis. The EarthScope program of the National Science Foundation has supported a transportable grid of magnetotelluric sensors across the United States. Deployed from 2006 to 2011 in the Pacific Northwest, the sensor grid is now being deployed across the north midwestern United States [Schultz, 2009].
In contrast, long-term measurements of the geoelectric field at observatories are much more sparsely distributed in geography. The Japanese Meteorological Agency has supported 1-second measurements of the geoelectric field at its three magnetic observatories since 1983; see Figure 2(b). Geoelectric measurements at observatories are supported by Germany’s GeoForschungsZentrum, the British Geological Survey, and France’s Institut de Physique du Globe de Paris. Analog geoelectric measurements were supported at the Tucson magnetic observatory from 1932 to 1942, but otherwise, very little long-term geoelectric monitoring has taken place in the United States. However, the USGS is considering a project for long-term 1-second resolution geoelectric monitoring at a few of its magnetic observatories.
Modeling and Mapping
Although some geoelectric monitoring is important, it is challenging to directly use geoelectric measurements to estimate induction hazards across continental or even small-scale regional geography. Storm time geoelectric fields realized at one site are not always well correlated with those at another site, a difficulty that is partly due to the localized complexity of lithospheric electrical conductivity [e.g., McKay and Whaler, 2006] and the relatively high conductivity of ocean water compared to the lithosphere. Therefore, a priority for induction hazard science is the development of three-dimensional models of Earth conductivity that have a spatial resolution similar to the spatial scale of regional electric power grids. Some progress on this front is being made in the United States. The EarthScope project has, for example, enabled modeling lithospheric conductivity under the Pacific Northwest [Meqbel et al., 2014].
Another factor affecting the induced geoelectric field is the spatial complexity of geomagnetic activity generated by magnetospheric-ionospheric electric currents. In principle, empirically parameterized maps of the temporal evolution of ground-level magnetic disturbance can be constructed by fitting basis function model parameters to ground magnetometer data [e.g., Pulkkinen et al., 2003], thus filling in the geography between magnetometer stations. Time-convolutional filtering of a magnetic activity map through a conductivity model can give a geoelectric field map [e.g., Thomson et al., 2009]. Geoelectric model accuracy can be established by comparison with geoelectric measurements, and indeed, a geoelectric modeling project can inform evaluations of the adequacy of the existing ground magnetometer network. In the end, estimates of the storm time geoelectric field need only be accurate enough to make operational hazard assessments and to enable mitigation of deleterious effects.
Space Weather Prediction
The space weather that drives magnetic storms originates at the Sun. Abrupt ejections of concentrated plasma from the solar corona can be detected using telescopes on the NASA–European Space Agency Solar and Heliospheric Observatory (SOHO) spacecraft. Coronal mass ejections typically take about 2 days to traverse the Sun–Earth distance; energetic ejections can cross the distance in as little as 18 hours. If an ejection is directed toward the Earth, then NOAA’s Space Weather Prediction Center (SWPC) will issue a prediction of the commencement time and intensity of a magnetic storm. From an upstream orbit between the Sun and Earth, NASA’s Advanced Composition Explorer (ACE) spacecraft monitors solar wind conditions, with transmitted data arriving at Earth 15 to 45 minutes in advance of an oncoming coronal mass ejection. Within the magnetosphere and above the ionosphere, several satellites provide in situ magnetic field monitoring of the Earth’s surrounding space environment.
Improved predictions of space weather require improved monitoring and physics-based modeling of the heliosphere and geospace environments. These developments will, in turn, facilitate predictions of ground-level magnetic disturbance, the accuracy of which can be measured against ground-based monitoring data [e.g., Pulkkinen et al., 2013]. Although forecasting induction hazards is still in its infancy and dedicated research efforts are required for further advancements, some new capabilities are being transitioned from testing at the Community Coordinated Modeling Center at NASA Goddard Space Flight Center into operations at NOAA SWPC [e.g., Pulkkinen et al., 2010].
Extreme Events
Over timescales longer than a few solar cycles, historical data from observatories reveal the statistical relationship between magnetic storm intensity and storm occurrence frequency [e.g., Riley, 2012]. This relationship can be used to forecast the future likelihood of magnetic storms across a range of intensities. Because extremely intense magnetic storms [e.g., see Pulkkinen et al., 2012] are, by definition, rare, retrospective statistical inferences end up being made from historical records of a small number of intense storms [e.g., Love, 2012].
Another approach for analyzing intense space weather events exploits the modern availability of solar wind data. For example, in July 2012 an extremely large coronal mass ejection event was recorded in situ by one of NASA’s Solar Terrestrial Relations Observatory (STEREO) satellites. This ejection was not Earth directed, but it provided researchers with an opportunity to study a space weather event that could have had deleterious consequences [Baker et al., 2013; Ngwira et al., 2013]. Together with historical data from ground observatories, this type of analysis informs scenario modeling of extreme events [e.g., Pulkkinen et al., 2012], and it is guiding the development of geomagnetic disturbance standards, such as those mandated by FERC.
Looking Forward
Induction hazard science is of increasing relevance for today’s modern, electricity-dependent society. Continued collaboration between government, academic, and private sectors will help maintain and expand real-time operational monitoring and modeling of the Earth and its surrounding space environment so that induction hazards can be better understood, evaluated, and predicted.
Acknowledgments
We thank C. A. Finn, A. Kelbert, J. McCarthy, and E. W. Worthington for reading a draft manuscript. We thank J. L. Gannon and W. S. Leith for useful conversations.
References
Baker, D. N., et al. (2008), Severe Space Weather Events: Understanding Societal and Economic Impacts, 144 pp., Natl. Acad. Press, Washington, D. C.
Baker, D. N., et al. (2013), A major solar eruptive event in July 2012: Defining extreme space weather scenarios, Space Weather, 11, 585–591, doi:10.1002/swe.20097.
Bolduc, L. (2002), GIC observations and studies in the Hydro-Québec power system, J. Atmos. Sol. Terr. Phys., 64, 1793–1802.
Kappenman, J. (2001), Advanced geomagnetic storm forecasting for the electric power industry, in Space Weather, Geophys. Monogr. Ser., vol. 125, edited by P. Song, H. J. Singer, and G. L. Siscoe, pp. 353–358, AGU, Washington, D. C., doi:10.1029/GM125p0353.
Love, J. J. (2012), Credible occurrence probabilities for extreme geophysical events: Earthquakes, volcanic eruptions, magnetic storms, Geophys. Res. Lett., 39, L10301, doi:10.1029/2012GL051431.
Love, J. J., and A. Chulliat (2013), An international network of magnetic observatories, Eos Trans. AGU, 94(42), 373–384, doi:10.1002/2013EO420001.
Love, J. J., and C. A. Finn (2011), The USGS Geomagnetism Program and its role in space weather monitoring, Space Weather, 9, S07001, doi:10.1029/2011SW000684.
McKay, A. J., and K. A. Whaler (2006), The electric field in northern England and southern Scotland: Implications for geomagnetically induced currents, Geophys. J. Int., 167, 613–625.
Meqbel, N., G. D. Egbert, P. E. Wannamaker, A. Kelbert, and A. Schultz (2014), Deep electrical resistivity structure of the northwestern U.S. derived from 3-D inversion of USArray magnetotelluric data, Earth Planet. Sci. Lett., 402, 290–304.
Ngwira, C., et al. (2013), Simulation of the 23 July 2012 extreme space weather event: What if the extremely rare CME was Earth directed?, Space Weather, 11, 671–679, doi:10.1002/2013SW000990.
Pirjola, R. (2002), Review on the calculation of surface electric and magnetic fields and of geomagnetically induced currents in ground-based technological systems, Surv. Geophys., 23, 71–90.
Pulkkinen, A., O. Amm, A. Viljanen, and Bear Working Group (2003), Separation of the geomagnetic variation field on the ground into external and internal parts using the spherical elementary current system, Earth Planets Space, 55, 117–129, doi:10.1002/2013SW000990.
Pulkkinen, A., et al. (2010), Solar shield: Forecasting and mitigating space weather effects on high-voltage power transmission systems, Nat. Hazards, 53, 333–345.
Pulkkinen, A., E. Bernaubeu, J. Eichner, C. Beggan, and A. W. P. Thomson (2012), Generation of 100-year geomagnetically induced current scenarios, Space Weather, 10, S04003, doi:10.1029/2011SW000750.
Pulkkinen, A., et al. (2013), Community-wide validation of geospace model ground magnetic field perturbation predictions to support model transition to operations, Space Weather, 11, 369–385, doi:10.1002/swe.20056.
Riley, P. (2012), On the probability of occurrence of extreme space weather events, Space Weather, 10, S02012, doi:10.1029/2011SW000734.
Schultz, A. (2009), EMScope: A continental scale magnetotelluric observatory and data discovery resource, Data Sci. J., 8, IGY6–IGY20.
Thomson, A. W. P., A. J. McKay, and A. Viljanen (2009), A review of progress in modelling of induced geoelectric and geomagnetic fields with special regard to induced currents, Acta Geophys., 57, 209–219.
Viljanen, A., A. Pulkkinen, O. Amm, R. Pirjola, T. Korja, and Bear Working Group (2004), Fast computation of the geoelectric field using the method of elementary current systems, Ann. Geophys., 22, 101–113.
Viljanen, A., et al. (2012), Continental scale modelling of geomagnetically induced currents, J. Space Weather Space Clim., 2, A17, doi:10.1051/swsc/2012017.
Yumoto, K., et al. (2012), ULTIMA: Array of ground-based magnetometer arrays for monitoring magnetospheric and ionospheric perturbations on a global scale, Abstract SM14A-01 presented at 2012 Fall Meeting, AGU, San Francisco, Calif., 9–13 Dec.
Author Information
Jeffrey J. Love and E. Joshua Rigler, Geomagnetism Program, U.S. Geological Survey, Denver, Colo.; email: jlove@usgs.gov; Antti Pulkkinen, NASA Goddard Space Flight Center, Greenbelt, Md.; and Christopher C. Balch, Space Weather Prediction Center, National Oceanic and Atmospheric Administration, Boulder, Colo.
Citation: Love, J. J., E. Joshua Rigler, A. Pulkkinen and C. C. Balch (2014), Magnetic storms and induction hazards, Eos Trans. AGU, 95(48), 445–446, doi:10.1002/2014EO480001.
Text not subject to copyright.
Except where otherwise noted, images are subject to copyright. Any reuse without express permission from the copyright owner is prohibited.
Text not subject to copyright.
Except where otherwise noted, images are subject to copyright. Any reuse without express permission from the copyright owner is prohibited.