Although the drivers of climate change and its consequences in polar regions are becoming better understood [Holland and Bitz, 2003] and well monitored [Serreze et al., 2002; Doran et al., 2002b], measuring the responses of polar landscapes to changing climate boundary conditions is challenging: Polar landscapes typically respond slowly to warming but abruptly to melting [Gooseff et al., 2011].
Time-lapse imaging has emerged as the premier method for capturing evidence of punctuated change to cryosphere landscapes [Balog, 2008; Ahn and Box, 2010; Dickson et al., 2013; Levy et al., 2013]. In polar environments, time-lapse imaging captures the dynamics of complex processes while also making the consequences of climate change relatable to the public. Here we highlight recent advances in time-lapse imaging for geosciences research in Antarctica.
Existing Monitoring
Most cryosphere landscape monitoring focuses on the collection of long-term climate and surface energy balance measurements [e.g., Fountain et al., 1999; Brown et al., 2000; Harris et al., 2001; Doran et al., 2002a; Smith et al., 2003; Romanovsky et al., 2010; Guglielmin et al., 2012]. These commonly combine mapping, geographic information system analyses, satellite mapping, ground-based imaging, and/or lidar scans [Barnhart and Crosby, 2013], but surface climatology data sets typically sample at a rate far in excess of the imaging or mapping rate. Thus, although meteorological data are typically continuous, measurements of landscape response are not. This mismatch motivated the acquisition of time-lapse imaging data sets that could be integrated with continuous meteorology data.
No off-the-shelf time-lapse system on the market permits the collection of time-lapse data with any camera or the integration of that imaging data with any time series data set collected from nearby environmental sensors. Thus, techniques had to be developed to integrate any time series data set, collected on any data logging platform, with any time-lapse imaging data set.
Time-Lapse Imaging Stations
Time-lapse imaging of polar landscapes presents several challenges, primarily low temperatures that inhibit battery performance and rugged terrain that severely limits accessibility to remote stations. These factors amplify the importance of developing an ultrastable imaging system because servicing equipment, particularly at stations that can only be accessed by helicopter, is often logistically impossible.
Commercial cameras can perform time-lapse image acquisition through a modification of their firmware. We have implemented the Canon Hack Development Kit (http://chdk.wikia.com/wiki/CHDK), which was developed by camera users to provide automated time-lapse functionality built into the system firmware. After modifying the firmware on new cameras, we field-installed them and recorded images during five field seasons in the McMurdo Dry Valleys (~77°S–78°S, 160°E–140°E).
Low-power (~3-volt) cameras can run through austral summer at 5-minute intervals using two photovoltaic panels (~7.5 watts) that charge two sealed 12-volt gel cell batteries. This power system performs well in extreme cold and is in compliance with environmental protocols governing fieldwork in Antarctica. Cameras operate until late March, providing observations that encompass the entirety of the peak melting season, and continue after science teams must return from the field. These image data (landscape response) are paired with environmental sensor data (climatic forcing), which are collected from stations within the camera’s field of view.
All image and sensor data are stored within a Linux file system for which software has been developed to generate side-by-side movies of climate measurements synchronized with time-lapse images (Figure 1) [Dickson et al., 2013; Levy et al., 2013]. A user accessing these data is prompted for key parameters (instrument, duration, interval, frame rate, etc.). The software queries the sensor data for the temporally closest measurement for each image on the basis of the image time stamp and plots the measurement next to the image. Thus, images and meteorology station recordings need not be precisely synchronous, as the software will find the closest possible match. This means that any image source can be paired with any tabular data file even if the two are not designed to be used in concert.
The linked time-lapse image/environmental sensor approach has led to new discoveries and key process associations. Two examples from the McMurdo Dry Valleys stand out—they demonstrate how integrated meteorologic stations and time-lapse data techniques can provide insight into glacial, fluvial, and permafrost processes.
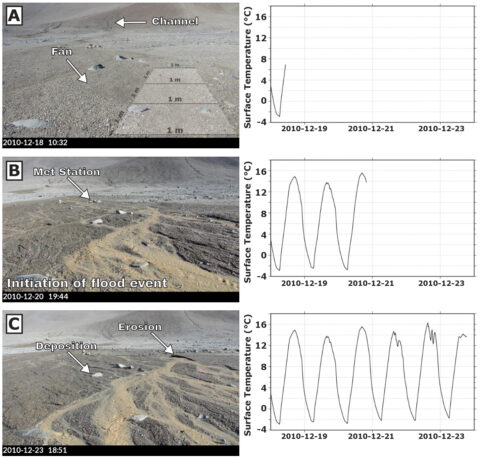
Brine Generation and Transport
The first example is from Don Juan Pond, located in Upper Wright Valley in the McMurdo Dry Valleys. The pond is the most saline natural body of water on Earth [Meyer et al., 1962], composed almost entirely of a calcium chloride brine. As such, it does not fully freeze and is a provocative site for determining the limits of extremophiles on Earth [Samarkin et al., 2010] and the potential for brines and primitive life on Mars [Burt and Knauth, 2003; Marchant and Head, 2007].
Long hypothesized to be sourced by deep groundwater [Harris and Cartwright, 1981], direct observations of inputs into the pond had previously not been published. During the 2009–2010 field campaign, time-lapse imaging provided direct evidence of discharge from seeps within the approximately 20-centimeter soil layer, correlated with daily temperature spikes, with no evidence of a deep groundwater contribution (see Movie 1 in the additional supporting information in the online version of this article) [Dickson et al., 2013].
This shallow brine activity is consistent with brine generation by deliquescence of calcium chloride salts [Wilson, 1979], which was also documented as time-lapse sequences recorded soil hydration correlated with increases in relative humidity (Movie 2 in the online version) [Dickson et al., 2013]. Time-lapse imaging in this analysis refined scientific understanding of brine generation and transport in polar environments, providing hypotheses for potential brine activity on Mars [McEwen et al., 2011].
Determining the Causes of Melting of a Buried Ice Sheet
Additionally, time-lapse imaging coupled with in situ meteorological observations in Garwood Valley, Antarctica, was used to determine the causes of accelerated loss of a buried ice sheet remnant emplaced in the valley bottom during the Pleistocene [Levy et al., 2013], about 20–30 thousand years ago. Time-lapse imaging showed that as the formerly buried ice was exposed to intense summer illumination at a dramatic ice cliff, melting and erosion of the frozen landscape accelerated.
While biannual lidar scans revealed that the melting rate of the ground ice was accelerating, the relative roles of melting versus debris avalanching and block failure from exposed ice cliffs were not clearly resolved. Likewise, because the stage of the Garwood River, which flows past the ice cliffs, is highly variable on seasonal and diurnal time scales [Levy et al., 2013], short visits to the field site were not sufficient to determine whether heat transfer from the river to the riverbank ice deposits was a significant factor.
Time-lapse imaging at the site revealed two major insights into the causes of accelerated ground ice melting in Garwood Valley (see Movie 3 in the online version). First, debris avalanches that remove the ice’s insulating sediment cover are activated thermally, occurring most often when the top of the underlying ice is already melting. This dusts the exposed ice with warm, dark sediment that further accelerates the melting along the full face of the ice cliffs. In addition, river level, which was thought to be warming the ice and causing melting, is usually well below the level at which ground ice melting and block failure occur in the riverbank, suggesting that insolation processes, rather than fluvial advection of heat, dominate the energy balance leading to rapid erosion of this ancient ice.
The Power of Observing Landscapes as They Evolve
Time-lapse imaging integrated with meteorological measurements provides unprecedented documentation of landscape processes as they evolve with changing climate conditions. The products generated enable fundamental scientific discoveries that are valuable to the scientific community and easily relatable to the public, including policy makers.
This versatility is motivating the development of related techniques, including stereo imaging with digital elevation model generation for three-dimensional time lapse (time-lapse topography), infrared time-lapse imaging for analysis of surface thermal evolution, and satellite communications with time-lapse stations for remote access to data. The value of time-lapse imaging is maximized when it is treated as a component of a larger monitoring network, focused on connecting the response of the Earth’s surface to the environmental processes that shape it.
Acknowledgments
We thank the dozens of colleagues who assisted with many field installations. In particular, guidance and support from Dave Marchant is greatly appreciated, as well as technical assistance from Brendan Hermalyn and Christoph Thomas. Logistical management from Raytheon Polar Services, Antarctic Support Contract, Petroleum Helicopters International, and Helicopters New Zealand greatly supported our efforts. This work was funded by the National Science Foundation Antarctic Science Division (Division of Polar Programs) through grants to J.W.H. (ANT-0739702), David R. Marchant (ANT-1144224), and J.S.L. (PLR-1343835 and PLR-1343649), which are gratefully acknowledged.
References
Ahn, Y., and J. E. Box (2010), Glacier velocities from time-lapse photos: Technique development and first results from the Extreme Ice Survey (EIS) in Greenland, J. Glaciol., 56(198), 723–734, doi:10.3189/002214310793146313.
Balog, J. D. (2008), Extreme Ice Survey, Eos Trans. AGU, 89(53), Fall Meet. Suppl., Abstract GC51A-0652.
Barnhart, T. B., and B. T. Crosby (2013), Comparing two methods of surface change detection on an evolving thermokarst using high-temporal-frequency terrestrial laser scanning, Selawik River, Alaska, Remote Sens., 5(6), 2813–2837.
Brown, J., K. M. Hinkel, and F. E. Nelson (2000), The Circumpolar Active Layer Monitoring (CALM) program: Research designs and initial results 1, Polar Geogr., 24(3), 166–258, doi:10.1080/10889370009377698.
Burt, D. M., and L. P. Knauth (2003), Electrically conducting, Ca-rich brines, rather than water, expected in the Martian subsurface, J. Geophys. Res., 108(E4), 8026, doi:10.1029/2002JE001862.
Dickson, J. L., J. W. Head, J. S. Levy, and D. R. Marchant (2013), Don Juan Pond, Antarctica: Near-surface CaCl2-brine feeding Earth’s most saline lake and implications for Mars, Sci. Rep., 3, 1166, doi:10.1038/srep01166.
Doran, P. T., C. P. McKay, G. D. Clow, G. L. Dana, A. G. Fountain, T. Nylen, and W. B. Lyons (2002a), Valley floor climate observations from the McMurdo Dry Valleys, Antarctica, 1986–2000, J. Geophys. Res., 107(D24), 4772, doi:10.1029/2001JD002045.
Doran, P. T., J. C. Priscu, W. B. Lyons, J. E. Walsh, A. G. Fountain, D. M. McKnight, D. L. Moorhead, R. A. Virginia, D. H. Wall, and G. D. Clow (2002b), Antarctic climate cooling and terrestrial ecosystem response, Nature, 415(6871), 517–520.
Fountain, A. G., W. B. Lyons, M. B. Burkins, G. L. Dana, P. T. Doran, K. J. Lewis, D. M. McKnight, D. L. Moorhead, A. N. Parsons, and J. C. Priscu (1999), Physical controls on the Taylor Valley ecosystem, Antarctica, BioScience, 49(12), 961–971.
Gooseff, M. N., A. Balser, W. B. Bowden, and J. B. Jones (2011), Effects of hillslope thermokarst in northern Alaska, Eos Trans. AGU, 90(4), 29–30, doi:10.1029/2009EO040001.
Guglielmin, M., M. R. Worland, and N. Cannone (2012), Spatial and temporal variability of ground surface temperature and active layer thickness at the margin of maritime Antarctica, Signy Island, Geomorphology, 155–156, 20–33, doi:10.1016/j.geomorph.2011.12.016.
Harris, C., W. Haeberli, D. Vonder Mühll, and L. King (2001), Permafrost monitoring in the high mountains of Europe: The PACE project in its global context, Permafrost Periglacial Processes, 12(1), 3–11, doi:10.1002/ppp.
Harris, H. J. H., and K. Cartwright (1981), Hydrology of the Don Juan Basin, Wright Valley, Antarctica, in Dry Valley Drilling Project, Antarct. Res. Ser., vol. 33, edited by L. D. McGinnis, pp. 161–184, AGU, Washington, D. C.
Holland, M. M., and C. M. Bitz (2003), Polar amplification of climate change in coupled models, Clim. Dyn., 21(3-4), 221–232, doi:10.1007/s00382-003-0332-6.
Levy, J. S., A. G. Fountain, J. L. Dickson, J. W. Head, M. Okal, D. R. Marchant, and J. Watters (2013), Accelerated thermokarst formation in the McMurdo Dry Valleys, Antarctica, Sci. Rep., 3, 2269, doi:10.1038/srep02269.
Marchant, D. R., and J. W. Head (2007), Antarctic dry valleys: Microclimate zonation, variable geomorphic processes, and implications for assessing climate change on Mars, Icarus, 192(1), 187–222.
McEwen, A. S., L. Ojha, C. M. Dundas, S. S. Mattson, S. Byrne, J. J. Wray, S. C. Cull, S. L. Murchie, N. Thomas, and V. C. Gulick (2011), Seasonal flows on warm Martian slopes, Science, 333(6043), 740–743.
Meyer, G. H., M. B. Morrow, O. Wyss, T. E. Berg, and J. L. Littlepage (1962), Antarctica: The microbiology of an unfrozen saline pond, Science, 138(3545), 1103–1104.
Romanovsky, V. E., S. L. Smith, and H. Christiansen (2010), Permafrost thermal state in the polar Northern Hemisphere during the international polar year 2007–2009: A synthesis, Permafrost Periglacial Processes, 21(2), 106–116.
Samarkin, V. A., M. T. Madigan, M. W. Bowles, K. L. Casciotti, J. C. Priscu, C. P. McKay, and S. B. Joye (2010), Abiotic nitrous oxide emission from the hypersaline Don Juan Pond in Antarctica, Nat. Geosci., 3, 341–344.
Serreze, M. C., D. H. Bromwich, M. P. Clark, A. J. Etringer, T. Zhang, and R. Lammers (2002), Large-scale hydro-climatology of the terrestrial Arctic drainage system, J. Geophys. Res., 108(D2), 8160, doi:10.1029/2001JD000919.
Smith, K. L., Jr., R. J. Baldwin, R. C. Glatts, T. K. Chereskin, H. Ruhl, and V. Lagun (2003), Weather, ice, and snow conditions at Deception Island, Antarctica: Long time-series photographic monitoring, Deep Sea Res., Part II, 50(10–11), 1649–1664, doi:10.1016/S0967-0645(03)00084-5.
Wilson, A. T. (1979), Geochemical problems of the Antarctic dry areas, Nature, 280, 205–208.
Author Information
James L. Dickson, Department of Earth, Environmental, and Planetary Science, Brown University, Providence, R.I.; email: [email protected]; Joseph S. Levy, Institute for Geophysics, University of Texas at Austin; and James W. Head, Department of Earth, Environmental, and Planetary Science, Brown University, Providence, R.I.
© 2014. American Geophysical Union. All rights reserved.
© 2014. American Geophysical Union. All rights reserved.