A translation of this article was made possible by a partnership with Planeteando. Una traducción de este artículo fue posible gracias a una asociación con Planeteando.
Ten years ago this month, the blowout and explosion aboard the Deepwater Horizon (DWH) oil rig killed 11 people and caused hundreds of millions of gallons of oil and natural gas to begin pouring into the Gulf of Mexico, a spill that eventually became the largest marine spill in U.S. history. In 2016, a federal district judge approved a $21 billion settlement with the companies involved—the largest settlement in U.S. history for damage done to natural resources—that included nearly $9 billion for the restoration of natural resources and the services they provide.
One result of this disaster was a substantial increase in the amount of research focused on oil spill science. Approximately 1 month after the spill began, for example, BP committed to providing an unprecedented $500 million over 10 years to fund independent research on the impacts of the spill. These funds established the Gulf of Mexico Research Initiative (GoMRI), which has supported a diverse array of research projects since 2010.
With this level of sustained and directed funding, the number of peer-reviewed papers published on oil spill science skyrocketed. A notable breakthrough that arose from this new body of research is that we now have a better grasp on how oil behaves, physically and chemically, once it enters the environment. In particular, the role of sunlight in photooxidizing floating surface oil, long discounted or overlooked, has taken on new precedence, and researchers now agree this role must be better accounted for in oil spill assessments and models.
Oil Weathers in Many Ways
When crude oil is spilled into the ocean, it undergoes a series of weathering processes, including dissolution, evaporation, emulsification, biodegradation, and photooxidation. Some of these processes relocate oil, whereas some transform it. For example, dissolution and evaporation transfer low–molecular weight hydrocarbons from oil into the water column and air, respectively, but they do not alter the chemical composition of these compounds. Emulsification is a process in which water is entrained by the oil, which changes the oil’s physical properties (in particular, it increases viscosity) but not its chemical properties. In contrast, biodegradation and photooxidation are transformative: They add oxygen to components in the oil, creating new compounds with different properties than those initially in the spilled oil.
Oil weathering processes have wide-ranging implications for ecosystem and human health, as well as for spill response operations.
These oil weathering processes have wide-ranging implications for ecosystem and human health, as well as for spill response operations. Dissolution of oil components into the water can facilitate microbial biodegradation while simultaneously increasing exposure of aquatic animals to harmful compounds. Evaporation of oil from the sea surface can expose governmental and industry oil spill first responders to toxic compounds. Moreover, evaporated oil can be photooxidized into secondary organic aerosols and ozone; this process negatively affected air quality along the Gulf Coast after the DWH spill [Middlebrook et al., 2012]. Photooxidation of floating oil makes it more difficult to clean up, contributing to oil residues that wash up on valuable and sensitive coastlines. All of these weathering processes are considered by first responders as they decide when and where to allocate precious resources to mitigate damages from the spilled oil.
As part of the ongoing GoMRI Synthesis and Legacy efforts, which aim to document and exploit scientific achievements and advances made over the past 10 years, we hosted a workshop with a group of experts on oil weathering at sea that included members of the federal government, academia, and industry. Attendees discussed all weathering processes, although for reasons presented below, the workshop focused mainly on photooxidation. The conclusions from this workshop were recently distilled into three take-home messages [Ward and Overton, 2020].
A Nonnegligible Process
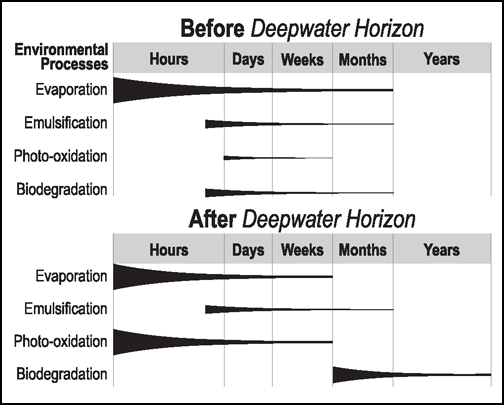
First, the rate and extent of photooxidation of oil floating on the sea surface after the DWH blowout far surpassed estimates based on early conceptual models of oil weathering. Before the spill, the consensus perspective across many subdisciplines of oil spill science was that evaporation, emulsification, and biodegradation were the most important weathering processes influencing the fate of oil spilled at sea (Figure 1). Photooxidation was widely considered to affect only the small fraction of light-absorbing aromatic compounds in oil [e.g., Garrett et al., 1998]. And photooxidation was not incorporated into mass balance assessments of spilled oil (e.g., the National Oceanic and Atmospheric Administration’s oil budget calculator), oil spill fate and transport models, or spill response guidance documents.
But more than a dozen studies published since 2010, making use of analytical tools from simple elemental analysis to state-of-the-art mass spectrometers, have documented the rapid and extensive photooxidation of oil floating on the sea surface during the DWH spill [Ward and Overton, 2020]. Ward et al. [2018a] estimated that within a week of surfacing, half of the floating oil was transformed by sunlight into new compounds with different physical and chemical properties. Photooxidation certainly was not a negligible weathering process as previously thought, and conceptual models have now been revised to reflect the importance of photochemical weathering (Figure 1) [National Research Council, 2019].
How Sunlight Oxidized All This Oil
The second take-home message focuses on how sunlight oxidized so much oil floating on the sea surface. There are two ways that oil photooxidation can occur: directly and indirectly (Figure 2). Direct photooxidation occurs when compounds in crude oil that absorb natural sunlight, such as polycyclic aromatic hydrocarbons, are oxidized. If this were the dominant pathway, oxidation would be limited to the tiny fraction of oil components that absorbs sunlight.
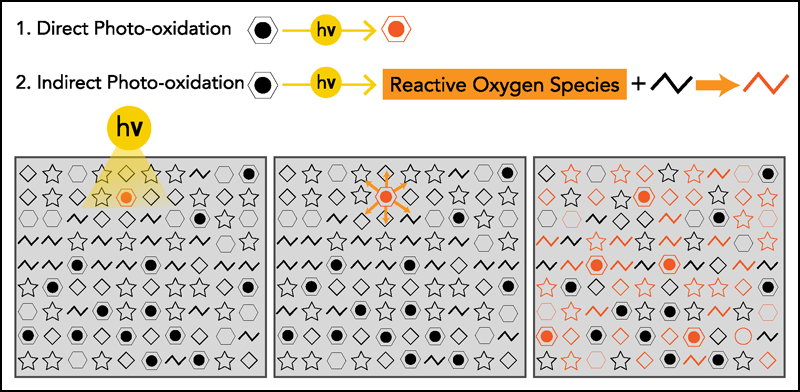
Indirect photooxidation is a little more complicated. When compounds in crude oil absorb sunlight, a wide range of reactive oxygen species are produced, including singlet oxygen, peroxy radicals, and hydroxyl radicals. These species can oxidize other compounds in oil, not just those that absorb light directly. Thus, if indirect photooxidation were the main oxidation pathway, a much larger fraction of spilled oil would be vulnerable to oxidation.
The oil spill research community now recognizes that a much larger fraction of spilled oil is vulnerable to oxidation by sunlight.
Prior to DWH, there was no consensus about whether direct or indirect photooxidation dominates. But with samples and resources in hand and access to newly developed analytical technologies, numerous field and laboratory studies have now documented the governing role that indirect photooxidation pathways play [e.g., Hall et al., 2013; Ruddy et al., 2014]. Again, half the floating oil from the DWH spill was photooxidized within a week [e.g., Aeppli et al., 2012; Ward et al., 2018a], much greater than the small percentage that absorbed light directly. By settling this long-standing debate about the main pathway of photooxidation, the oil spill research community now recognizes that a much larger fraction of spilled oil is vulnerable to oxidation by sunlight.
Adding Photochemistry to Models and Response Plans
The third take-home message relates to incorporating photochemistry into oil spill models that predict the many fates of spilled oil on the sea surface over space and time, information that is critical to effective response contingency planning. Historically, such models neglected to consider the Sun’s effects on oil properties. Moreover, the performance of tools used in response to oil spills, such as chemical dispersants, was rarely evaluated with respect to photooxidized oil. Instead, dispersants were traditionally evaluated with respect to evaporation and emulsification because these processes alter the viscosity of the spilled oil.
The oil spill response community is now starting to acknowledge the prudence of incorporating sunlight-driven processes into oil spill models and response contingency planning because sunlight alters both the physical and chemical properties of crude oil at the sea surface. There is synergy between photochemical weathering and emulsification. Sunlight produces surface-active compounds that reside at the oil-water interface and that promote the formation of highly viscous and stable emulsions, which are very challenging to disperse.
This synergy was hypothesized roughly 40 years ago [Thingstad and Pengerud, 1982; Overton et al., 1980], but it could not be tested because of analytical constraints at the time. In a win for the iterative and long-arching nature of science, once the research community overcame these constraints, the hypothesis was confirmed [Zito et al., 2020]. Still, this synergy is not fully captured in oil spill models for surface spills because of the lack of data to quantify this process, giving researchers pause about the accuracy of model predictions.
Changes to the chemical composition of floating oil once released into the environment also affect the performance of chemical dispersants. In principle, these dispersants work by breaking up floating surface oil into droplets that disperse into the water column and thus reduce the amount of oil that reaches sensitive coastal ecosystems (Figure 3). But only a few days of sunlight exposure, which changes how floating oil interacts with chemical dispersants, may reduce dispersant effectiveness by 30% [Ward et al., 2018b]. Modeling efforts comparing the time that oil floated at sea prior to treatment with dispersants versus oil photooxidation rates further indicate that a considerable fraction of dispersant applications during the DWH spill may have targeted photooxidized oil that was not easily dispersed [Ward et al., 2018b]. These impacts of sunlight exposure on chemical dispersant effectiveness at the sea surface were likely not reported by earlier studies simply because photochemical oxidation was not perceived to affect a significant fraction of the floating spilled oil.
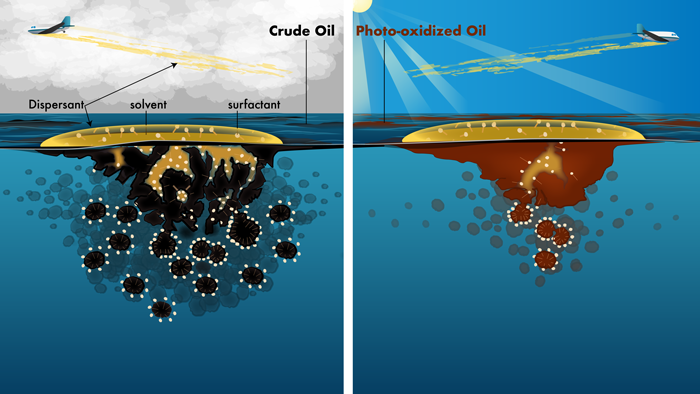
Where Are We Now?
The 10-year anniversary of the DWH spill provides an opportunity to reflect not only on our improved understanding of photochemical weathering of oil at sea but also on why it took a devastating environmental disaster to make such progress.
There are four key reasons why the DWH spill sparked the advancement in knowledge described above:
1. A Unique Sample Set. Oil floated on the sea surface for 102 days, allowing researchers ample time to coordinate sampling campaigns that yielded an extremely rare and valuable set of oil samples. These samples provided opportunities to validate laboratory-based predictions about the rates, relative importance, and controls of oil weathering processes under natural field conditions.
2. Sustained Funding. The sustained funding provided by GoMRI and other sources allowed researchers time to follow up on early findings. Early studies about the extent of oxidation [e.g., Aeppli et al., 2012; Lewan et al., 2014; Ruddy et al., 2014] laid foundations for later studies of oxidation rates and pathways [Ward et al., 2018a, 2019; Niles et al., 2019] and their potential impacts on fate and transport models and response operations [Ward et al., 2018b; Zito et al., 2020].
Satellite-based remote sensing technologies provided estimates of oil film surface area and thickness throughout the 102-day period of surface oiling, a key parameter for estimating rates of photooxidation.
3. Technological Breakthroughs. Advances in technology proved critical. Satellite-based remote sensing technologies provided estimates of oil film surface area and thickness throughout the 102-day period of surface oiling [MacDonald et al., 2015], a key parameter for estimating rates of photooxidation [Ward et al., 2018a]. Comprehensive two-dimensional gas chromatography coupled with flame ionization detection helped determine the precursors of photooxidation, which proved to be mainly compounds that do not absorb light directly [Hall et al., 2013]. On the other side of the reaction scheme, Fourier transform ion cyclotron resonance mass spectrometry helped determine the products of photooxidation [e.g., Ruddy et al., 2014; Niles et al., 2019], confirming that indirect processes governed oxidation. Last, novel separation technologies [Clingenpeel et al., 2017] allowed researchers to isolate and identify oil components that are produced by sunlight, partition to the oil-water interface, and promote emulsification [Zito et al., 2020], corroborating Thingstad and Pengerud’s [1982] decades-old hypothesis.
4. Diversified Expertise. The DWH spill sparked unprecedented interdisciplinary collaborations and insights into the photochemical weathering of oil at the sea surface. The expertise represented in these collaborations was wide-ranging, including petroleum and environmental chemists, modelers and oil spill response scientists, and biogeochemists and isotope geochemists. This interdisciplinary approach, in which foundational information learned from basic science was applied to fill long-standing knowledge gaps, was undoubtedly a formula for success. Moreover, interdisciplinary approaches taken to study the DWH spill, such as tracing oil photooxidation using stable oxygen isotopes [Ward et al., 2019], will likely lead to a more complete understanding of the cycling of other reduced forms of carbon, like organic pollutants and natural organic matter.
Where We Go from Here
Perhaps now more than ever, we have a prime opportunity to continue advancing oil spill science with sustained and directed research.
Our vastly improved understanding of photochemical weathering of floating oil at sea is a clear example of the accomplishments made in oil spill science in the past 10 years, and we have learned so much more about spill dynamics, biodegradation, ecosystem responses, and other issues. Perhaps now more than ever, we have a prime opportunity to continue advancing oil spill science with sustained and directed research. The workforces and laboratories are primed and ready, the cross-disciplinary connections are established, and findings from the past 10 years provide a road map for future research priorities. These priorities include (1) establishing the applicability of findings from the DWH spill to other scenarios, such as spills at different water depths (i.e., surface versus deepwater) or involving different oil types (i.e., light to heavy, sweet to sour) or in different locations (e.g., temperate versus high-latitude waters); (2) assessing the impact of photooxidation on the effectiveness of chemical agents used in oil spill response operations (e.g., herders and surface-washing agents) other than dispersants; and (3) developing empirical data sets to incorporate photochemical processes into oil spill fate, transport, and response operation models.
Despite widespread calls to curb global petroleum use and notwithstanding the current reduced consumption and drop in demand tied to the COVID-19 pandemic, global demand is expected to climb steadily [International Energy Agency, 2019]. Even in a scenario in which policies are adopted to curb demand, demand is predicted not to plateau until the 2030s. It follows that oil spills at sea will continue to happen and perhaps even accelerate with a shift in offshore oil production from shallow-water resources (<125 meters depth) to more technically challenging deepwater (125–1,500 meters) and ultradeepwater (>1,500 meters) resources. Let’s not slow down or deemphasize oil spill research just because a decade has passed since 2010. The more knowledge gaps we fill now about the fate, transport, and impacts of oil spills, the better prepared we will be to respond to the next big spill.
References
Aeppli, C., et al. (2012), Oil weathering after the Deepwater Horizon disaster led to the formation of oxygenated residues, Environ. Sci. Technol., 46, 8,799–8,807, https://doi.org/10.1021/es3015138.
Clingenpeel, A. C., et al. (2017), Fractionation of interfacial material reveals a continuum of acidic species that contribute to stable emulsion formation, Energy Fuels, 31, 5,933–5,939, https://doi.org/10.1021/acs.energyfuels.7b00490.
Garrett, R. M., et al. (1998), Photooxidation of crude oils, Environ. Sci. Technol., 32, 3,719–3,723, https://doi.org/10.1021/es980201r.
Hall, G. J., et al. (2013), Oxygenated weathering products of Deepwater Horizon oil come from surprising precursors, Mar. Pollut. Bull., 75, 140–149, https://doi.org/10.1016/j.marpolbul.2013.07.048.
International Energy Agency (2019), World Energy Outlook 2019, Organ. for Econ. Coop. and Dev., Paris, https://doi.org/10.1787/caf32f3b-en.
Lewan, M. D., et al. (2014), Asphaltene content and composition as a measure of Deepwater Horizon oil spill losses within the first 80 days, Org. Geochem., 75, 54–60, https://doi.org/10.1016/j.orggeochem.2014.06.004.
MacDonald, I. R., et al. (2015), Natural and unnatural oil slicks in the Gulf of Mexico, J. Geophys. Res. Oceans, 120, 8,364–8,380, https://doi.org/10.1002/2015JC011062.
Middlebrook, A. M., et al. (2012), Air quality implications of the Deepwater Horizon oil spill, Proc. Natl. Acad. Sci. U. S. A., 109(50), 20,280–20,285, https://doi.org/10.1073/pnas.1110052108.
National Research Council (2019), The Use of Dispersants in Marine Oil Spill Response, Natl. Acad. Press, Washington, D.C.
Niles, S. F., et al. (2019), Molecular-level characterization of oil-soluble ketone/aldehyde photo-oxidation products by Fourier transform ion cyclotron resonance mass spectrometry reveals similarity between microcosm and field samples, Environ. Sci. Technol., 53, 6,887–6,894, https://doi.org/10.1021/acs.est.9b00908.
Overton, E. B., et al. (1980), Photochemical oxidation of IXTOC I oil, in Proceedings of a Symposium on Preliminary Results from the September 1979 Researcher/Pierce IXTOC-I Cruise: Key Biscayne, Florida, June 9–10, 1980, pp. 341–383, Off. of Mar. Pollut. Assess., Boulder, Colo.
Ruddy, B. M., et al. (2014), Targeted petroleomics: Analytical investigation of Macondo well oil oxidation products from Pensacola Beach, Energy Fuels, 28, 4,043–4,050, https://doi.org/10.1021/ef500427n.
Thingstad, T., and B. Pengerud (1982), The formation of “chocolate mousse” from Statfjord crude oil and seawater, Mar. Pollut. Bull., 14, 214–216, https://doi.org/10.1016/0025-326X(83)90254-0.
Ward, C. P., and E. B. Overton (2020), How the 2010 Deepwater Horizon spill reshaped our understanding of crude oil photochemical weathering at sea: A past, present, and future perspective, Environ. Sci. Processes Impacts, https://doi.org/10.1039/D0EM00027B, in press.
Ward, C. P., et al. (2018a), Partial photochemical oxidation was a dominant fate of Deepwater Horizon surface oil, Environ. Sci. Technol., 52, 1,797–1,805, https://doi.org/10.1021/acs.est.7b05948.
Ward, C. P., et al. (2018b), Photochemical oxidation of oil reduced the effectiveness of aerial dispersants applied in response to the Deepwater Horizon spill, Environ. Sci. Technol. Lett., 5, 226–231, https://doi.org/10.1021/acs.estlett.8b00084.
Ward, C. P., et al. (2019), Oxygen isotopes (δ18O) trace photochemical hydrocarbon oxidation at the sea surface, Geophys. Res. Lett., 46, 6,745–6,754, https://doi.org/10.1029/2019GL082867.
Zito, P., et al. (2020), Sunlight-induced molecular progression of oil into oxidized oil soluble species, interfacial material, and dissolved organic matter, Energy Fuels, 34, 4,721–4,726, https://doi.org/10.1021/acs.energyfuels.9b04408.
Author Information
Collin P. Ward ([email protected]) and Christopher M. Reddy, Department of Marine Chemistry and Geochemistry, Woods Hole Oceanographic Institution, Mass.; and Edward B. Overton, College of the Coast and Environment, Louisiana State University, Baton Rouge
Citation:
Ward, C. P.,Reddy, C. M., and Overton, E. B. (2020), Why sunlight matters for marine oil spills, Eos, 101, https://doi.org/10.1029/2020EO143427. Published on 28 April 2020.
Text © 2020. The authors. CC BY-NC-ND 3.0
Except where otherwise noted, images are subject to copyright. Any reuse without express permission from the copyright owner is prohibited.