Solar climate intervention, also known as solar radiation modification, is an approach intended to mitigate the impacts of climate change by reducing the amount of solar energy that the Earth system traps. It sits alongside three other plausible responses to climate risk: emission cuts and decarbonization, atmospheric carbon dioxide (CO2) removal, and adaptation to a changing climate.
Unlike the other approaches, solar climate intervention (SCI), which comprises various techniques, aims to modify Earth’s radiation budget—the amounts and balance of solar energy that Earth absorbs and reflects—directly. Implementing SCI means either decreasing inbound solar (shortwave) radiation by reflecting it back into space before it is absorbed or increasing the amount of outbound terrestrial (longwave) radiation.
Potential methods of SCI include stratospheric aerosol injection (SAI), marine cloud brightening, cirrus cloud thinning, surface albedo modification, and space-based methods involving, for example, mirrors (Figure 1). At present, the potential efficacy and risks of implementing these approaches to reduce climate change are highly uncertain and likely depend on how they are implemented.
The Geoengineering Modeling Research Consortium (GMRC) was founded to coordinate SCI modeling research and to identify and resolve relevant issues with physical models, especially where existing climate research is unlikely to do so. Here we synthesize 2 years of GMRC meetings and research, and we offer specific recommendations for future model development.
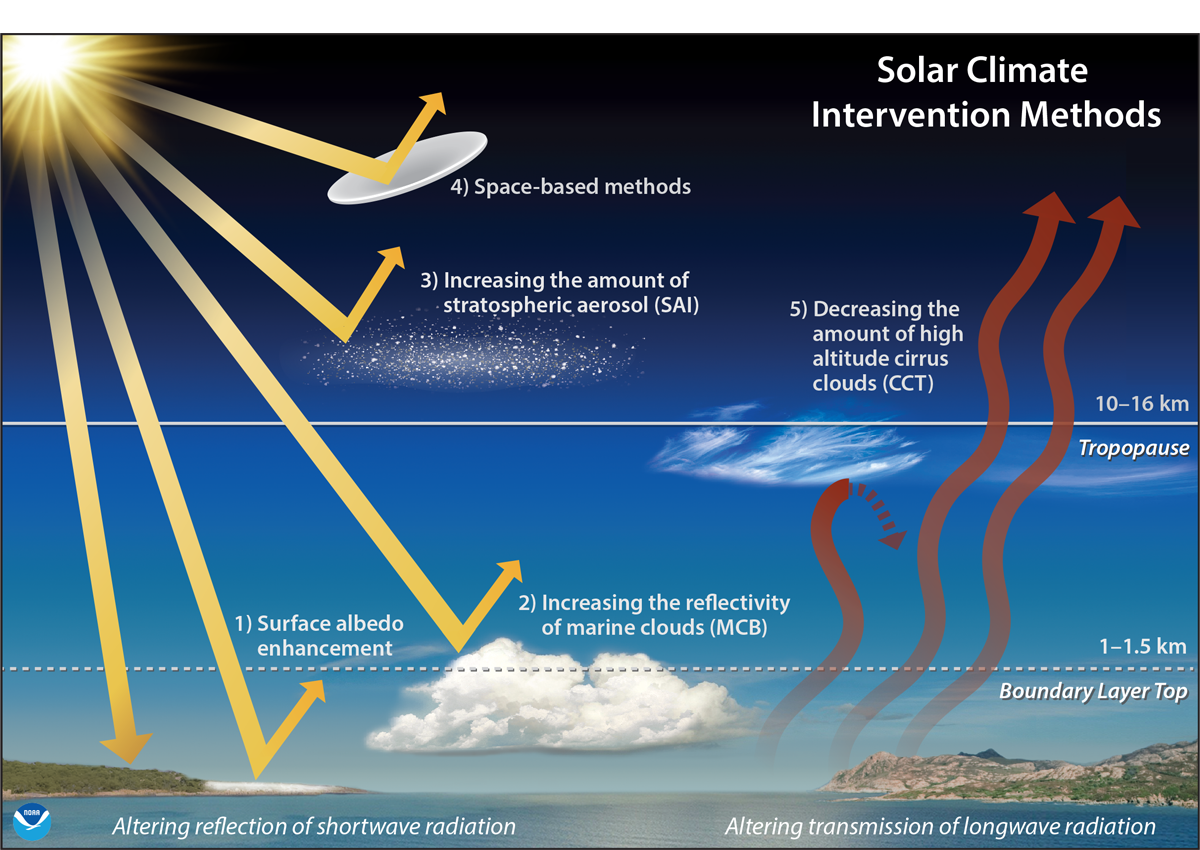
Current Climate Models and SCI Simulations
SCI research is an engineering application of Earth system science focused on developing the capacity to reduce the magnitude of climate change. SCI strategies are commonly investigated using global climate models (GCMs). However, these models are most frequently used to study the consequences of anthropogenic emissions (e.g., of CO2), and they are not optimized to represent SCI’s deployment and effects.
Thus, critical processes in SCI simulation, including fundamental physical, chemical, and biogeophysical processes that occur at subgrid scales (i.e., finer than the resolution of GCMs), are missing or poorly represented by these models (Figure 2). For example, most models used to study stratospheric aerosol intervention cannot resolve the physics and chemistry of an injected aerosol plume in the stratosphere, or its subsequent interactions with cloud processes.
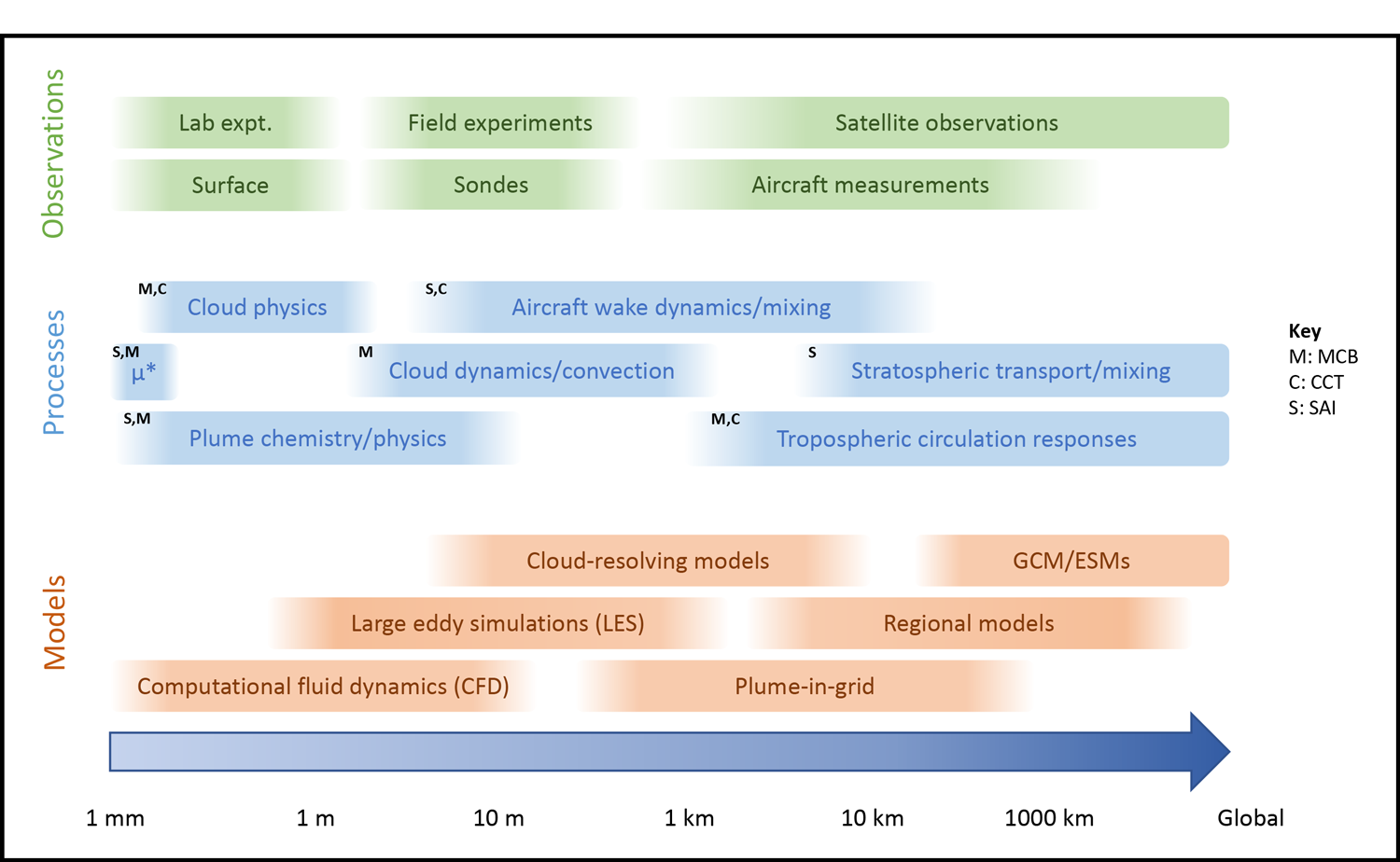
Broader uncertainties also come into play: Different climate models estimate different radiative forcings (human-induced changes in the radiative budget), resulting in different simulated climate impacts. These differences persist even when the models use a common, crude representation of the effects of SCI, such as a uniform increase in stratospheric aerosol optical depth (i.e., a quantifiable decrease in the “transparency” of the stratosphere).
Meanwhile, in some cases (e.g., cirrus cloud thinning), significant uncertainties remain concerning even the fundamental physics of relevant processes. Because of these uncertainties, even agreement among multiple models does not imply scientific confidence in the result. Targeted and coordinated modeling efforts are needed to quantify and reduce these uncertainties.
Priorities for SCI Research
Solar climate intervention methods all involve local-scale perturbations that affect the regional and global climate through chains of processes operating at increasingly large spatial scales.
The forms of SCI most discussed in the literature include SAI, marine cloud brightening, and cirrus cloud thinning. These methods all involve local-scale perturbations that affect the regional and global climate through chains of processes operating at increasingly large spatial scales. The range of scales is too great to be represented by any single numerical model or accurately characterized by any single observational approach (Figure 2).
Predictions of SCI’s net climate impacts are therefore especially sensitive to the accuracy of parameterizations used to characterize processes and variables that occur at or below the scale of typical GCM grid cells. Furthermore, some of the large-scale climate perturbations that SCI efforts may produce are likely to be outside the range of parameters (such as microphysical parameters controlling the behavior of stratospheric aerosols) for which we have observations, such that we are left extrapolating otherwise reliable parameterizations into unknown conditions.
As an example of this problem, we have no direct, real-world examples of how atmospheric circulation—and therefore weather patterns—would respond to a sustained increase in solar reflection. Thus, our simulations of its effects are extrapolations based on our knowledge of “related” phenomena, such as volcanic eruptions in which particles enter the stratosphere in a large, brief event and then fall out of the stratosphere over a few years without replenishment.
To resolve this mismatch of scales, we need a systematic effort to identify whether relevant, small-scale processes are well understood physically; well represented by high-resolution, local-scale modeling; and accurately parameterized for use in global-scale models.
Imperfect Analogues
More direct observations are needed to better understand and more accurately model small-scale processes that are important for geoengineering.
A long history of observational atmospheric science has informed models used to study geoengineering, but more direct observations are needed to better understand and more accurately model small-scale processes that are important for geoengineering yet still poorly constrained.
For example, volcanic eruptions help to constrain simulations of large-scale changes to stratospheric aerosol loading and have been discussed as an analogue for geoengineering. However, volcanic aerosols provide little data suitable for modeling the likely behavior of localized emissions resulting from proposed SAI strategies, such as the deployment of aerosols in aircraft plumes.
Another potential analogue comes from observations of interactions between clouds and aerosol emissions from ships. Although these observations provide some information on how marine clouds are likely to respond to brightening, much about cloud responses remains uncertain because of the complexity of these systems. It is also difficult to isolate the effects of aerosol changes, because aerosol emissions from ships are poorly constrained and because these effects are often closely tied to local meteorology. More fundamental research into the dynamic and thermodynamic responses of marine clouds to aerosol perturbations and into how ambient and added aerosols compete for cloud water is needed.
Simulations of cirrus cloud thinning are even less well constrained, necessitating research to investigate questions concerning what makes an effective ice nucleus—that is, what types of solid particles are most likely to form a coating of ice, seeding the formation of ice crystals. Research is also needed to investigate the degree to which existing cirrus clouds are formed homogeneously (ice crystals forming without solid seed particles) or heterogeneously (ice crystals forming around dust and aerosol particles).
Testing Modeled Processes Against Observations
Existing observational data sets can be used to test model accuracy when representing some key processes relevant to SCI mechanisms while at the same time helping to determine what other key processes remain insufficiently constrained. We need to identify both the capability of existing models to reproduce existing observations and where more observations are needed to address knowledge gaps. We can do this by performing systematic simulations that explore the ranges of conditions covered by current modeling approaches.
Such an effort will also provide insights into both internal variability in Earth system conditions (e.g., natural changes in marine conditions associated with El Niño) and true uncertainty in current modeling approaches. This separation between variability and uncertainty is a complex issue with many unknowns. To efficiently use computer resources, a single group using only one or a few models could perform an initial investigation to establish whether a given approach yields a significant response, following which a larger effort by groups such as the Geoengineering Model Intercomparison Project (GeoMIP) would be warranted.
Bridging the Resolution Gap
To improve parameterizations in global models, a coordinated effort involving multiple research groups is needed to bridge the “resolution gaps” evident in Figure 2. Increasing the resolution of global models is useful but not sufficient. We must identify the resolution at which the simulated behavior of each process converges to a common solution (e.g., droplet-level modeling of a cloud compared to global cloud parameterizations) and whether this common solution agrees with available empirical observations. This effort will help to determine physical situations or phenomena for which resolution-aware approaches (e.g., resolution-dependent scaling factors, nested gridding, plume-in-grid methods, and adaptive mesh refinement) might be sufficient to re-create observations, as opposed to those for which a new parameterization or subgrid representation is necessary.
A priority is to develop approaches that explicitly, but efficiently, represent plume-scale processes, such as an SAI plume or cirrus seeding track, in global-scale models. Results from such efforts will need to be compared with existing observations where available, and new observations designed to address the knowledge gaps most relevant to SCI may be needed to verify these approaches’ behavior.
Responses Across Scales
Each local-scale change will drive larger-scale responses, involving a wide array of feedbacks, many of which are poorly represented in large-scale models.
The bottom-up view presented above addresses only half of the problem. Each local-scale change will drive larger-scale responses. There is considerable evidence that regional forcing—whether conventional or SCI specific—leads to a global response through multiple teleconnections in all components of the Earth system. A global response can occur, for example, through atmospheric pathways, such as local heating perturbations exciting atmospheric waves. Changes in ocean circulation or land feedbacks like windblown desert sand or dust storms can also produce effects on a global scale. A key element of this problem is that it involves a wide array of feedbacks, many of which are poorly represented in large-scale models.
Existing observations can be used, to a limited extent, to constrain the scope and scale of simulated teleconnections and feedbacks relevant to SCI, but this approach relies on there being clear natural proxies for SCI forcing. No such proxy exists for the regional and global-scale responses to significant perturbations in clouds imposed in targeted regions. Even for SAI, volcanoes provide an imperfect proxy. Investigations, including simplified forcing simulations, are therefore needed to identify SCI-relevant teleconnections and feedbacks that relate regional forcing to global change.
Connecting Models to the Real World
Even though the GMRC’s focus is on coordinating modeling efforts and improving models rather than on acquiring real-world observations, the two efforts must be connected. In some cases, models may be improved by incorporating better representations of processes that are already well constrained by observations. In others, model uncertainty is dominantly caused by a lack of empirical knowledge of processes.
There is, for example, a clear path to improving representations of stratospheric aerosols and marine cloud brightening, which are reasonably well constrained by observations, through the use of multiscale modeling. On the other hand, a major source of uncertainty in predictions of cirrus thinning is the lack of both data and methods needed to estimate the prevalence of homogeneous nucleation in cirrus cloud formation.
We will also need experiments conducted in the field to study effects of SCI-related perturbations specifically and test model representations of small-scale processes. These experiments must fill scientific knowledge gaps and uncertainties (some of which can already be identified) that will be difficult to resolve without observations of controlled perturbations. Such dedicated SCI experiments could be conducted at small enough scales so as not to incur significant regional or global effects. For example, aerosols could be added to a single cirrus cloud or to marine clouds over an area of, say, several square kilometers or injected into a single stratospheric aerosol plume.
Strategies for Moving Ahead
Model-based projections for the change in rainfall as a result of SCI could include an estimate of the probability of staying within limits that avoid either droughts or monsoons.
A goal of GMRC is to identify the observations that are most needed to reduce uncertainty in model predictions of SCI responses across spatial scales. Progress toward this goal will require collaboration with observational researchers to collect these observations and estimate empirical uncertainty for relevant parameterizations. It will also require developing structured modeling or model intercomparison exercises for SCI methods that can quantify empirical uncertainties—using an agreed upon set of metrics—that are important for predicting weather and climate impacts of interest to policymakers. For example, model-based projections for the change in rainfall as a result of SCI could include an estimate of the probability of staying within limits that avoid either droughts or monsoons.
Last, continued SCI research will require focused conversations among researchers modeling climate and climate impacts as well as others in the broader scientific community and with decision-makers in government and civil society. These conversations should identify the potential SCI impacts that most urgently need to be quantified and which SCI scenarios are most realistic.
If we pursue these strategies, researchers and policymakers can make substantial and rapid progress in studying and understanding the SCI methods that might eventually be needed to mitigate the risks of climate change facing humanity in the present and into the future.
Acknowledgments
This article was coauthored by the entire Geoengineering Modeling Research Consortium (GMRC) steering committee. This committee is composed of Karen Rosenlof (NOAA), Jean-François Lamarque (National Center for Atmospheric Research), and the five listed authors. Rosenlof and Lamarque contributed equally to this article but agreed not to be included in the author list to satisfy Eos coauthorship limits. This publication is (partially) funded by the Joint Institute for the Study of the Atmosphere and Ocean under NOAA cooperative agreement NA15OAR4320063, contribution 2020-1125. The steering committee also thanks the entire GMRC for their contributions.
Author Information
Sebastian Eastham ([email protected]), Laboratory for Aviation and the Environment and Joint Program on the Science and Policy of Global Change, Massachusetts Institute of Technology, Cambridge; also at John A. Paulson School of Engineering and Applied Sciences, Harvard University, Cambridge, Mass.; Sarah Doherty, Cooperative Institute for Climate, Ocean, and Ecosystem Studies, University of Washington, Seattle; David Keith, John A. Paulson School of Engineering and Applied Sciences, Harvard University, Cambridge, Mass.; Jadwiga H. Richter, Climate and Global Dynamics Laboratory, National Center for Atmospheric Research, Boulder, Colo.; and Lili Xia, Department of Environmental Sciences, Rutgers University, New Brunswick, N.J.
Citation: Eastham, S., S. Doherty, D. Keith, J. H. Richter, and L. Xia (2021), Improving models for solar climate intervention research, Eos, 102, https://doi.org/10.1029/2021EO156087. Published on 19 March 2021.
Text © 2021. The authors. CC BY-NC-ND 3.0
Except where otherwise noted, images are subject to copyright. Any reuse without express permission from the copyright owner is prohibited.