Gas hydrates are solid compounds that form as freezing water traps gases, most commonly methane, in a crystal structure under low temperature and high pressure. They are found beneath the seafloor of every ocean, as well as beneath deep freshwater lakes and Arctic permafrost. The amount of methane in gas hydrates rivals that in traditional deposits of natural gas—more than 6 trillion tons are trapped beneath the seafloor alone.
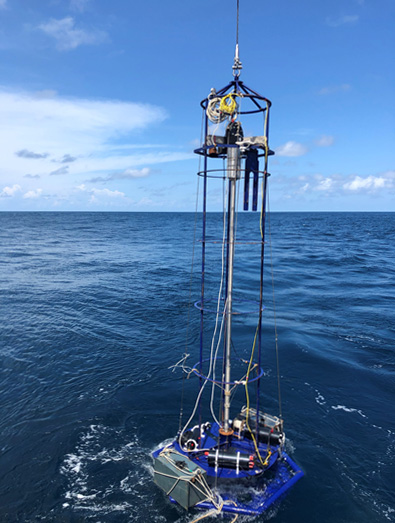
Gas hydrates may be a rich source of natural gas, but the extraction industry surrounding them is still in its infancy. The process of extracting gas hydrates from their subterranean environment is complex, as the compounds tend to disintegrate when removed. The technology relies on depressurizing the material while it is still on the seafloor and then channeling the gas to the surface. Although Japan and China have successfully tested methane hydrate extraction procedures in the South China Sea and elsewhere, the process remains far from commercially viable.
Some of the chief obstacles to widespread extraction of gas hydrates, however, are geological and not technological. Removing methane from its cold, high-pressure environment can release an enormous amount of gas—methane in hydrates is concentrated at 164 times its volume at atmospheric level. As the hydrate dissociates into gas and fluids, it can trigger sudden deformations of the seafloor, such as mud volcanoes, pockmarks, and submarine landslides, which in turn can lead to disasters like tsunamis.
To be able to manage these geohazards, developing a way to detect dissociating gas hydrates in the seafloor is essential. As a result, Chaoqi Zhu, a geologist at Ocean University of China and Qingdao National Laboratory for Marine Science and Technology, and his colleagues set out to examine temperature and pressure changes during gas hydrate dissociation and how these changes influence deformations of the seafloor. Their experiment, published in the journal Energy Reports, aims to help forecast geohazards caused by gas hydrate dissociation.
Bringing the Ocean Floor into the Laboratory
Zhu and his colleagues re-created a scene that normally transpires hundreds or thousands of meters below sea level in their laboratory. Because creating the temperature and pressure conditions that methane hydrates require is difficult in a laboratory setting, the researchers used an artificial gas hydrate called R11, which can form below 8.5°C at atmospheric pressure.
As researchers monitored pressure on the seafloor, they observed a curious pattern of a slow increase followed by a sudden drop that resembled breathing.
Using a hair dryer, they blew warm air on the hydrate to destabilize it and watched the gas expand in snaking pipes and tiny gas bubbles. As they monitored the pressure, they observed a curious pattern of a slow increase followed by a sudden drop that resembled breathing.
“We could liken the seafloor to a balloon. When we blow up the balloon, its volume increases, driven by pressure,” wrote Zhu in an email.
Similarly, pressure in the seafloor increases gradually during hydrate dissociation and may form uplift structures such as mud volcanoes. When the pressure reaches a critical level, some of the fluid pathways will connect to seawater, resulting in a sudden release of pressure that can trigger subsidence, pockmarks, and even landslides. “The seafloor goes up and down, driven by hydrate-related pressure, resembling the volume change of our thorax or lungs with breathing in and out,” added Zhu.
Beatrice Castellani, an assistant professor at the University of Perugia, Italy, often creates hydrates herself in laboratory reactors but was not involved in the new research. She said that although the study was innovative, she had doubts about how well an artificial hydrate can represent natural processes.
“We usually try to approach the natural conditions inside the reactor. We try to reproduce hydrates with the same sediment and use pure methane or a mixture similar to natural gas,” said Castellani.
“It’s very difficult to monitor hydrate dissociation processes because you would have to look at the subseafloor all the time, and that’s just not possible.”
Although hydrate dissociation in an artificial laboratory setup may not be an exact replica of what happens at the bottom of the ocean, from a geologist’s perspective the results are still significant, said other experts like Judith Elger, a geophysicist at GEOMAR Helmholtz Centre for Ocean Research in Kiel, Germany, who was not involved in the study: Finally, we can observe the behavior of gas and fluid during dissociation in real time.
“The approach of the study is unique, as it uses these lab experiments,” said Elger. “It’s very difficult to actually monitor these [hydrate dissociation] processes because you would have to look at the subseafloor all the time, and that’s just not possible.”
The Challenges of Commercial Hydrate Exploitation
In the scramble for a share of the depleting pool of fossil fuels, gas hydrates will likely become a major nonrenewable energy source. Several countries—including China, India, Japan, Korea, and the United States—have set their eyes on developing commercial hydrate production and are currently testing exploitation methods. With China and Japan leading the way, it is possible that hydrate exploitation will reach commercial levels between 2030 and 2040.
According to Zhenyuan Yin, an assistant professor at Tsinghua Shenzhen International Graduate School in China, a lot more work must be done to bring hydrate exploitation safely to a commercial level. As well as increasing the efficiency of the process, we must deal with the issue of geohazards, said Yin, who was not involved in the research.
Zhu and his colleagues are working on bringing their analysis to offshore production tests. “The pressure monitoring saves time for people to cope with imminent geohazards during the upcoming gas hydrate commercial exploitation,” he said.
—Fanni Daniella Szakal (@FanniDaniella), Science Writer