Are the processes that generate planetary habitability in our solar system common or rare elsewhere? Answering this fundamental question poses an enormous challenge.
For example, observing Earth-analogue exoplanets—that is, Earth-sized planets orbiting within the habitable zone of their host stars—is difficult today and will remain so even with the next-generation James Webb Space Telescope (JWST) and large-aperture ground-based telescopes. In coming years, it will be much easier to gather data on—and to test hypotheses about the processes that generate and sustain habitability using—“Earth cousins.” These small-radius exoplanets lack solar system analogues but are more accessible to observation because they are slightly bigger or slightly hotter than Earth.
Here we discuss four classes of exoplanets and the investigations of planetary materials that are needed to understand them (Figure 1). Such efforts will help us better understand planets in general and Earth-like worlds in particular.
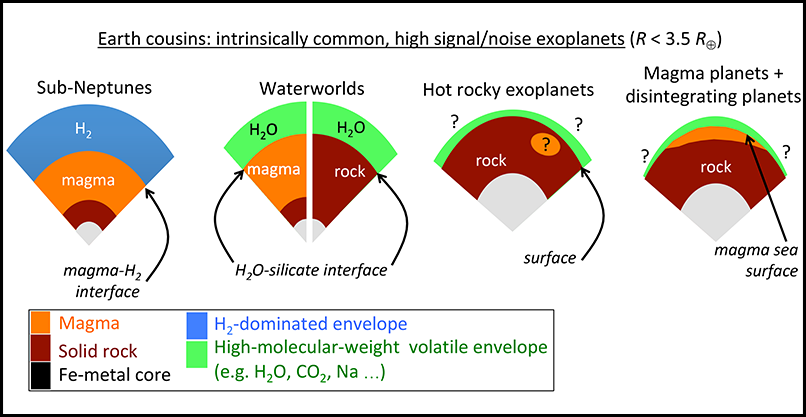
What’s in the Air?
Atmospheres are now routinely characterized for Jupiter-sized exoplanets. And scientists are acquiring constraints for various atmospheric properties of abundant smaller worlds.
On exoplanets, the observable is the atmosphere. Atmospheres are now routinely characterized for Jupiter-sized exoplanets. And scientists are acquiring constraints for various atmospheric properties of smaller worlds (those with a radius R less than 3.5 Earth radii R⨁), which are very abundant [e.g., Benneke et al., 2019; Kreidberg et al., 2019]. Soon, observatories applying existing methods and new techniques such as high-resolution cross-correlation spectroscopy will reveal even more information.
For these smaller worlds, as for Earth, a key to understanding atmospheric composition is understanding exchanges between the planet’s atmosphere and interior during planet formation and evolution. This exchange often occurs at interfaces (i.e., surfaces) between volatile atmospheres and condensed (liquid or solid) silicate materials. For many small exoplanets, these interfaces exhibit pressure-temperature-composition (P–T–X) regimes very different from Earth’s and that have been little explored in laboratory and numerical experiments. To use exoplanet data to interpret the origin and evolution of these strange new worlds, we need new experiments exploring the relevant planetary materials and conditions.
Studying Earth cousin exoplanets can help us probe the delivery and distribution of life-essential volatile species—chemical elements and compounds like water vapor and carbon-containing molecules, for example, that form atmospheres and oceans, regulate climate, and (on Earth) make up the biosphere. Measuring abundances of these volatiles on cousin worlds that orbit closer to their star than the habitable zone is relatively easy to do. These measurements are fundamental to understanding habitability because volatile species abundances on Earth cousin exoplanets will help us understand volatile delivery and loss processes operating within habitable zones.
For example, rocky planets now within habitable zones around red dwarf stars must have spent more than 100 million years earlier in their existence under conditions exceeding the runaway greenhouse limit, suggesting surface temperatures hot enough to melt silicate rock into a magma ocean. So whether these worlds are habitable today depends on the amount of life-essential volatile elements supplied from sources farther from the star [e.g., Tian and Ida, 2015], as well as on how well these elements are retained during and after the magma ocean phase.
Different types of Earth cousin exoplanets offer natural solutions that can ease volatile detection.
Volatiles constitute a small fraction of a rocky planet’s mass, and quantifying their abundance is inherently hard. However, different types of Earth cousin exoplanets offer natural solutions that can ease volatile detection. For example, on planets known as sub-Neptunes, the spectroscopic fingerprint of volatiles could be easier to detect because of their mixing with lower–molecular weight atmospheric species like hydrogen and helium. These lightweight species contribute to more puffed-up (expanded) and thus more detectable atmospheres. Hot, rocky exoplanets could “bake out” volatiles from their interiors while also heating and puffing up the atmosphere, which would make spectral features more visible. Disintegrating rocky planets may disperse their volatiles into large, and therefore more observable, comet-like tails.
Let’s look at each of these examples further.
Unexpected Sub-Neptunes
About 1,000 sub-Neptune exoplanets (radius of 1.6–3.5 R⨁) have been confirmed. These planets, which are statistically about as common as stars, blur the boundary between terrestrial planets and gas giants.
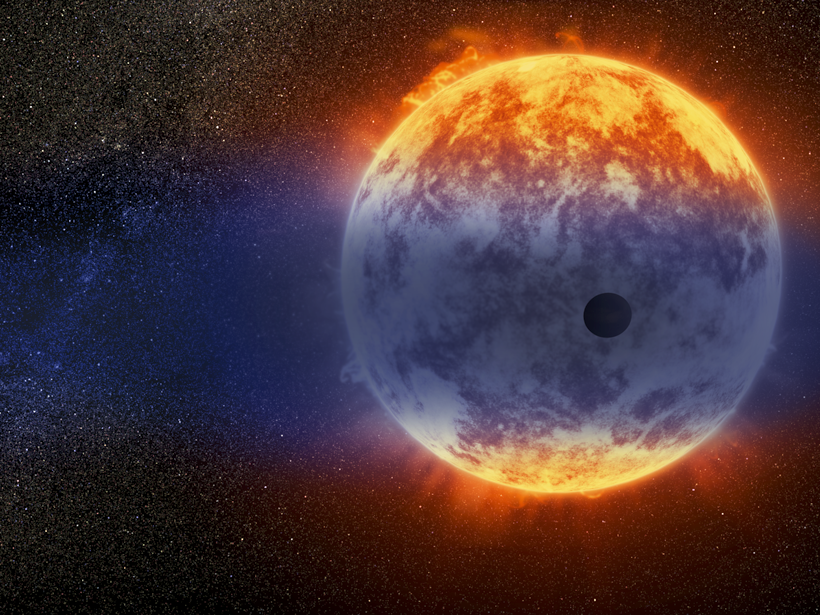
Strong, albeit indirect, evidence indicates that the known sub-Neptunes are mostly magma by mass and mostly atmosphere by volume (for a review, see Bean et al. [2021]). This evidence implies that an interface occurs, at pressures typically between 10 and 300 kilobars, between the magma and the molecular hydrogen (H2)-dominated atmosphere on these planets. Interactions at and exchanges across this interface dictate the chemistry and puffiness of the atmosphere. For example, water can form and become a significant fraction of the atmosphere, leading to more chemically complex atmospheres.
Improved molecular dynamics calculations are needed to quantify the solubilities of gases and gas mixtures in realistic magma ocean compositions (and in iron alloys composing planetary cores, which can also serve as reservoirs for volatiles) over a wider range of pressures and temperatures than we have studied until now. These calculations should be backed up by laboratory investigations of such materials using high-pressure instrumentation like diamond anvil cells. These calculations and experiments will provide data to help determine the equation of state (the relationship among pressure, volume, and temperature), transport properties, and chemical kinetics of H2-magma mixtures as they might exist on these exoplanets.
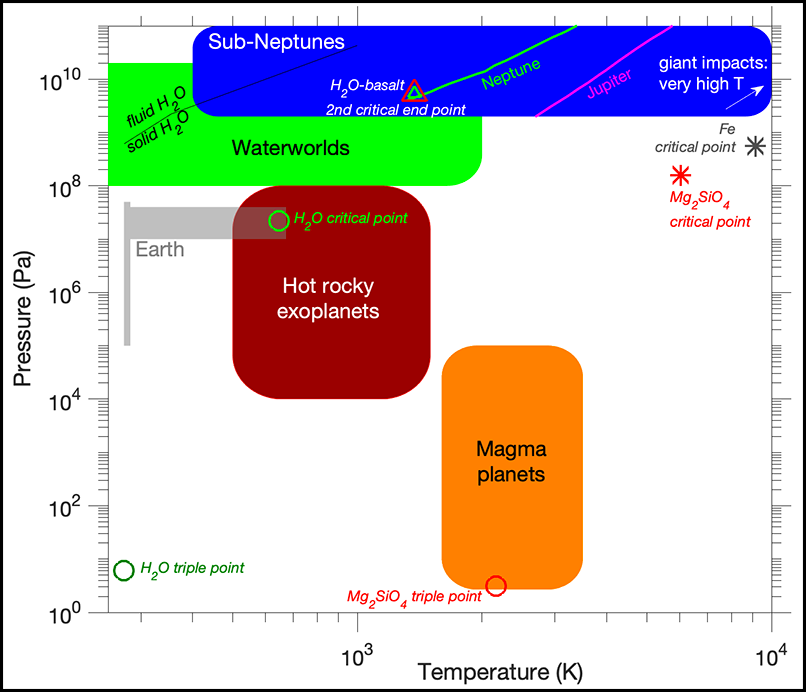
Because sub-Neptunes are so numerous, we cannot claim to understand the exoplanet mass-radius relationship in general (in effect, the equation of state of planets in the galaxy) without understanding interactions between H2 and magma on sub-Neptunes. To understand the extent of mixing between H2, silicates, and iron alloy during sub-Neptune assembly and evolution, we need more simulations of giant impacts during planet formation [e.g., Davies et al., 2020], as well as improved knowledge of convective processes on these planets. Within the P-T-X regimes of sub-Neptunes, full miscibility between silicates and H2 becomes important (Figure 2).
Beyond shedding light on the chemistry and magma-atmosphere interactions on these exoplanets, new experiments may also help reveal the potential for and drivers of magnetic fields on sub-Neptunes. Such fields might be generated within both the atmosphere and the magma.
Hot and Rocky
Hot, rocky exoplanets experience high fluxes of atmosphere-stripping ultraviolet photons and stellar wind, but whether they retain life-essential elements like nitrogen, carbon, and sulfur is unknown.
From statistical studies, we know that most stars are orbited by at least one roughly Earth-sized planet (radius of 0.75–1.6 R⨁) that is irradiated more strongly than our Sun’s innermost planet, Mercury. These hot, rocky exoplanets, of which about a thousand have been confirmed, experience high fluxes of atmosphere-stripping ultraviolet photons and stellar wind. Whether they retain life-essential elements like nitrogen, carbon, and sulfur is unknown.
On these hot, rocky exoplanets—and potentially on Venus as well—atmosphere-rock or atmosphere-magma interactions at temperatures too high for liquid water will be important in determining atmospheric composition and survival. But these interactions have been only sparingly investigated [Zolotov, 2018].
Many metamorphic and melting reactions between water and silicates under kilopascal to tens-of-gigapascal pressures are already known from experiments or are tractable using thermodynamic models. However, less well understood processes may occur in planets where silicate compositions and proportions are different than they are on Earth, meaning that exotic rock phases may be important. Innovative experiments and modeling that consider plausible exotic conditions will help us better understand these planets. Moreover, we need to conduct vaporization experiments to probe whether moderately volatile elements are lost fast enough from hot, rocky planets to form a refractory lag and reset surface spectra.
Exotic Water Worlds?
Water makes up about 0.01% of Earth’s mass. In contrast, the mass fraction of water on Europa, Ceres, and the parent bodies of carbonaceous chondrite meteorites is some 50–3,000 times greater than on Earth. Theory predicts that such water-rich worlds will be common not only in habitable zones around other stars but even in closer orbits as well. The JWST will be able to confirm or refute this theory [Greene et al., 2016].
If we could descend through the volatile-rich outer envelope of a water world, we might find habitable temperatures at shallow depths [Kite and Ford, 2018]. Some habitable layers may be cloaked beneath H2. Farther down, as the atmospheric pressure reaches 10 or more kilobars, we might encounter silicate-volatile interfaces featuring supercritical fluids [e.g., Nisr et al., 2020] and conditions under which water can be fully miscible with silicates [Ni et al., 2017].
We still need answers to several key questions about these worlds. What are the equilibria and rates of gas production and uptake for rock-volatile interfaces at water world “seafloors”? Can they sustain a habitable climate? With no land, and thus no continental weathering, can seafloor reactions supply life-essential nutrients? Do high pressures and stratification suppress the tectonics and volcanism that accelerate interior-atmosphere exchange [Kite and Ford, 2018]?
Relative to rock compositions on Earth, we should expect exotic petrologies on water worlds.
As for the deep interiors of Titan and Ganymede in our own solar system, important open questions include the role of clathrates (compounds like methane hydrates in which one chemical component is enclosed within a molecular “cage”) and the solubility and transport of salts through high-pressure ice layers.
Experiments are needed to understand processes at water world seafloors. Metamorphic petrologists are already experienced with the likely pressure-temperature conditions in these environments, and exoplanetary studies could benefit from their expertise. Relative to rock compositions on Earth, we should expect exotic petrologies on water worlds—for example, worlds that are as sodium rich as chondritic meteorites. Knowledge gained through this work would not only shed light on exoplanetary habitability but also open new paths of research into studying exotic thermochemical environments in our solar system.
Magma Seas and Planet Disintegration
Some 100 confirmed rocky exoplanets are so close to their stars that they have surface seas of very low viscosity magma. The chemical evolution of these long-lived magma seas is affected by fractional vaporization, in which more volatile materials rise into the atmosphere and can be relocated to the planet’s dark side or lost to space [e.g., Léger et al., 2011; Norris and Wood, 2017], and perhaps by exchange with underlying solid rock.
Magma planets usually have low albedos, reflecting relatively little light from their surfaces. However, some of these planets appear to be highly reflective, perhaps because their surfaces are distilled into a kind of ceramic rich in calcium and aluminum. One magma planet’s thermal signature has been observed to vary from month to month by a factor of 2 [Demory et al., 2016], implying that it undergoes a global energy balance change more than 10,000 times greater than that from anthropogenic climate change on Earth. Such large swings suggest that fast magma ocean–atmosphere feedbacks operate on the planet.
To learn more about the chemical evolution and physical properties of exoplanet magma seas, we need experiments like those used to study early-stage planet formation, which can reveal information about silicate vaporization and kinetics under the temperatures (1,500–3,000 K) and pressures (10−5 to 100 bars) of magma planet surfaces.
Exoplanets and exoplanetesimals that stray too close to their stars are destroyed—about five such cases have been confirmed. These disintegrating planets give geoscientists direct views of exoplanetary silicates because the debris tails can be millions of kilometers long [van Lieshout and Rappaport, 2018]. For disintegrating planets that orbit white dwarf stars, the debris can form a gas disk whose composition can be reconstructed [e.g., Doyle et al., 2019].
To better read the signals of time-variable disintegration, we need more understanding of how silicate vapor in planetary outflows condenses and nucleates, as well as of fractionation processes at and above disintegrating planets’ surfaces that may cause observed compositions in debris to diverge from the bulk planet compositions.
Getting to Know the Cousins
Investigating Earth cousins will illuminate the processes underpinning habitability in our galaxy and reveal much that is relevant for understanding Earth twins.
In the near future, new observatories like JWST and the European Space Agency’s Atmospheric Remote-sensing Infrared Exoplanet Large-survey (ARIEL, planned for launch in 2029) will provide new data. When they do, and even now before they come online, investigating Earth cousins will illuminate the processes underpinning habitability in our galaxy and reveal much that is relevant for understanding Earth twins.
From sub-Neptunes, for example, we can learn about volatile delivery processes. From hot, rocky planets, we can learn about atmosphere-interior exchange and atmospheric loss processes. From water worlds, we can learn about nutrient supplies in exoplanetary oceans and the potential habitability of these exotic environments. From disintegrating planets, we can learn about the interior composition of rocky bodies.
Laboratory studies of processes occurring on these worlds require only repurposing and enhancing existing experimental facilities, rather than investing in entire new facilities. From a practical standpoint, the scientific rewards of studying Earth cousins are low-hanging fruit.
Acknowledgments
We thank the organizers of the AGU-American Astronomical Society/Kavli workshop on exoplanet science in 2019.
References
Bean, J., et al. (2021), The nature and origins of sub-Neptune size planets, J. Geophys. Res. Planets, 126(1), e2020JE006639, https://doi.org/10.1029/2020JE006639.
Benneke, B., et al. (2019), A sub-Neptune exoplanet with a low-metallicity methane-depleted atmosphere and Mie-scattering clouds, Nat. Astron., 3, 813–821, https://doi.org/10.1038/s41550-019-0800-5.
Davies, E. J., et al. (2020), Silicate melting and vaporization during rocky planet formation, J. Geophys. Res. Planets, 125(1), e2019JE006227, https://doi.org/10.1029/2019JE006227.
Demory, B.-O., et al. (2016), Variability in the super-Earth 55 Cnc e, Mon. Notices R. Astron. Soc., 455, 2,018–2,027, https://doi.org/10.1093/mnras/stv2239.
Doyle, A., et al. (2019), Oxygen fugacities of extrasolar rocks: Evidence for an Earth-like geochemistry of exoplanets, Science, 366, 356–358, https://doi.org/10.1126/science.aax3901.
Greene, T. P., et al. (2016), Characterizing transiting exoplanet atmospheres with JWST, Astrophys. J., 817, 17, https://doi.org/10.3847/0004-637X/817/1/17.
Kite, E. S., and E. Ford (2018), Habitability of exoplanet waterworlds, Astrophys. J., 864, 75, https://doi.org/10.3847/1538-4357/aad6e0.
Kreidberg, L., et al. (2019), Absence of a thick atmosphere on the terrestrial exoplanet LHS 3844b, Nature, 573, 87–90, https://doi.org/10.1038/s41586-019-1497-4.
Léger, A., et al. (2011), The extreme physical properties of the CoRoT-7b super-Earth, Icarus, 213, 1–11, https://doi.org/10.1016/j.icarus.2011.02.004.
Ni, H., et al. (2017), Supercritical fluids at subduction zones: Evidence, formation condition, and physicochemical properties, Earth Sci. Rev., 167, 62–71, https://doi.org/10.1016/j.earscirev.2017.02.006.
Nisr, C., et al. (2020), Large H2O solubility in dense silica and its implications for the interiors of water-rich planets, Proc. Natl. Acad. Sci. U. S. A., 117, 9747, https://doi.org/10.1073/pnas.1917448117.
Norris, C. A., and B. J. Wood (2017), Earth’s volatile contents established by melting and vaporization, Nature, 549, 507–510, https://doi.org/10.1038/nature23645.
Tian, F., and S. Ida (2015), Water contents of Earth-mass planets around M dwarfs, Nat. Geosci., 8, 177–180, https://doi.org/10.1038/ngeo2372.
van Lieshout, R., and S. A. Rappaport (2018), Disintegrating rocky exoplanets, in Handbook of Exoplanets, pp. 1,527–1,544, Springer, Cham, Switzerland, https://doi.org/10.1007/978-3-319-55333-7_15.
Zolotov, M. (2018), Chemical weathering on Venus, in Oxford Research Encyclopedia of Planetary Science, edited by P. Read et al., Oxford Univ. Press, Oxford, U.K., https://doi.org/10.1093/acrefore/9780190647926.013.146.
Author Information
Edwin Kite ([email protected]), University of Chicago, Ill.; Laura Kreidberg, Max Planck Institute for Astrophysics, Heidelberg, Germany; Laura Schaefer, Stanford University, Calif.; Razvan Caracas, École Normale Supérieure de Lyon, France; and Marc Hirschmann, University of Minnesota, Twin Cities, Minneapolis
Citation:
Kite, E., L. Kreidberg, L. Schaefer, R. Caracas, and M. Hirschmann (2021), “Earth cousins” are new targets for planetary materials research, Eos, 102, https://doi.org/10.1029/2021EO159473. Published on 10 June 2021.
Text © 2021. The authors. CC BY-NC-ND 3.0
Except where otherwise noted, images are subject to copyright. Any reuse without express permission from the copyright owner is prohibited.