Most of the massive ice sheet that covers roughly four fifths of Greenland melts at the surface in summer. As long as the ice sheet regains its mass in the winter, this is not catastrophic. However, if the ice sheet melted entirely, sea levels would rise by more than 7 meters, with obvious and severe consequences for human civilization.
Not surprisingly, scientists are working hard to determine if and when the ice sheet will transition (or if it has already transitioned) from a stable state to a net mass loss state. The impact of increasing greenhouse gas levels on the Greenland ice sheet (GrIS) depends on many complex and interacting factors.
One is the ice sheet’s albedo—the fraction of incoming solar radiation that is reflected from the surface of the ice sheet. Indeed, scientists have determined that net solar radiation reaching the ice is the largest contributor to the energy balance driving melting [e.g., van den Broeke et al., 2011]. Despite the crucial role of albedo in energy balance, we have yet to quantify the role of the different processes driving it. Such an understanding is crucial to determining the past behavior of the GrIS and projecting its future contribution to sea level rise.
Scientists seeking to quantify how much various factors contribute to ice sheet albedo face numerous challenges. These include intrinsic limitations in current observational capabilities (e.g., spatial and radiometric resolution of currently available spaceborne sensors) and limitations on how accurately surface energy balance models handle ice sheet albedo. Moreover, the sparseness in space and time of in situ observations of quantities such as impurity concentrations, biological processes, and grain growth impedes our ability to separate their respective contributions to broadband albedo (integrated over the entire spectrum).
Darkening: A Complex Suite of Processes
The GrIS albedo has declined substantially in recent decades [Tedesco et al., 2014], attracting interest from both the scientific community and news media. Media reports, many using the term “darkening,” have emphasized that an increase in light-absorbing impurities, particularly black carbon and dust, in Greenland snow could be responsible for the observed albedo reduction. The idea is that such impurities absorb incoming sunlight, heating up and accelerating snow and ice melt.
Surface impurities can indeed reduce albedo in the visible part of the electromagnetic spectrum—light with wavelengths from 400 to 700 nanometers—and make snow and ice appear darker to our eyes. Images of “dirty” snow or ice are therefore visually appealing and can be powerful communication tools.
At infrared wavelengths, other powerful means of albedo reduction become important.
However, half the solar energy Earth receives is at near-infrared wavelengths (between 700 and 2500 nanometers), which is invisible to our eyes. At these wavelengths, other powerful means of albedo reduction become important. Hence, a comprehensive assessment of GrIS darkening must account for all processes that contribute to albedo reduction. Important factors include snow grain size, the impurity content of snow, biological activity, exposure of bare ice, formation of melt pools, and the combined effects and feedbacks associated with all of these factors.
We briefly discuss these processes below. We assert that each is potentially significant enough that the scientific community must quantify its role in GrIS darkening. Because each process responds positively to warming (i.e., albedo decreases as warming increases), they are all likely to become increasingly important in the future.
Snow Grain Growth: An Invisible Effect on Albedo
Soon after snow falls, its grains begin to change shape and size. Snow grains become rounded. Large grains grow at the expense of small grains, so the average grain radius increases with snowpack age.
At near-infrared wavelengths, coarse-grained snow has lower albedo than fine-grained snow. Warming accelerates this snow aging process, leading to further albedo reduction. In melting snow, grains become further rounded and clump into clusters. Thus, there is a positive feedback between warming, snow aging, increased solar absorption, and reduced albedo.
Since the reduction of albedo by grain growth is confined to the near infrared, it is mostly invisible to our eyes. This means that a clean snowpack (one with no impurities) that has melted at the surface can have a lower broadband albedo than a cold snowpack with impurities, despite the fact that the clean snowpack might still appear brighter to our eyes.
For pure snow, grain growth from new snow (grains around 0.1 millimeter in radius) to old melting snow (radius around 1 millimeter) can reduce broadband albedo by around 10%. By comparison, adding 20 parts per billion of black carbon, a concentration typical of those that scientists have found in the top layer of melting GrIS snow in the percolation zone, reduces albedo by only 1% to 2%.
Dirt on the Surface
Light-absorbing impurities such as black carbon, organic carbon, and dust are deposited on the GrIS from the atmosphere. Over most of the central GrIS, however, the impurity content in cold snow is quite low—about an order of magnitude lower than in the low-altitude Arctic. This is because of the high elevation of the central GrIS: Pollutant fluxes are mostly confined to lower altitudes.
To date, the amount of black carbon in the region of the GrIS that is losing mass, known as the ablation zone, has not been quantified. Measurements here are complicated by complex terrain and by the difficulty of separating black carbon from other particles (e.g., dust) in the snow. Although some scientists have hypothesized that dust may be darkening the ablation zone in southwest Greenland in particular, nobody has quantified the relative roles of black carbon, dust, and other darkening agents in these regions.
Additionally, insoluble impurities concentrate at the surface when snow melts since meltwater percolates down through the snowpack more efficiently than do particulates [e.g., Doherty et al., 2013]. This further lowers the albedo and enhances melting, leading to more consolidation [Flanner et al., 2007].
This positive feedback gives rise to high concentrations of impurities in the ablation zones in particular. Because of this feedback loop, in a snowpack that has partially melted, it is not possible to distinguish whether elevated impurity concentrations caused enhanced melting or resulted from enhanced melting (or both).
Life on Snow and Ice
Organisms on the ice sheet’s surface also reduce the ice’s albedo. Green, pink, purple, brown, and black pigmented algae can grow in melting snow and ice. On ice, cryoconite (a mixture of dust, pebbles, soot, and microbes) also absorbs sunlight. Furthermore, microbes can bind to particulates like black carbon, retaining them at the surface in higher concentrations than in the parent snow and ice.
As with black carbon, nobody has quantified the magnitude of this source of darkening. As the climate warms and melt seasons lengthen, biological habitats will expand, and their contribution to darkening will likely increase [Benning et al., 2014].
Bare Ice and Water Pools
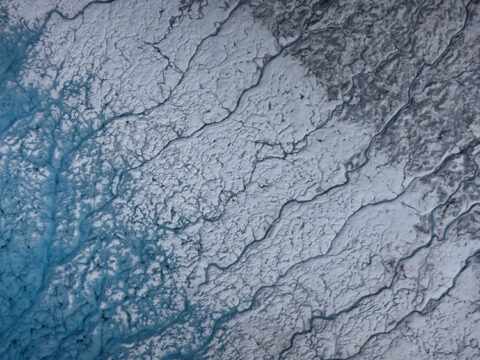
The exposure of bare ice and development of surface meltwater pools also reduces surface albedo, primarily in the near-infrared but also at visible wavelengths. The total albedo of clean glacier ice is around 60%, compared to 72% for clean melting snow.
Ice albedo decreases further, to between 20% and 50%, when it has high concentrations of impurities, as is common in the ablation zone. The albedo of melt pools is even lower, typically reaching values of 20%–30%.
Meltwater lakes on the ice sheet’s margin have also expanded substantially inland to higher elevations with warming, decreasing albedo over sizable areas of the ice sheet [Howat et al., 2013].
Recommendations
To determine exactly what causes the GrIS albedo to change, the scientific community must first quantify the contributions made by all of the processes described above. Currently, no such assessment exists. Instead, black carbon’s role in reducing visible albedo has attracted most of the attention. We need to communicate the other important processes involved in albedo reduction to both scientists and the general public.
We need to communicate the important processes involved in albedo reduction to scientists and the general public.
Remote sensing data can provide large-scale information, at high temporal resolution, on processes occurring on the ice sheet surface. Measuring and attributing albedo variations by means of satellite retrievals is challenging [Warren, 2013], however, because of the relatively low concentration of impurities on the surface of the GrIS and the relatively coarse spatial and radiometric resolution of satellite observations, among other things. We therefore need airborne campaigns or improved spaceborne sensors to collect finer spectral and spatial remote sensing data sets.
We also need in situ measurements in the GrIS’s ablation zone that can distinguish the relative contributions of different impurity types (e.g., black carbon, dust, algae) to albedo reduction as well as models that accurately simulate the GrIS surface energy balance and mass balance. For example, regional climate models need to refine the modeling of snow grain size and exposure of bare ice and need to include impurities and biological activity in their albedo schemes.
A Critical Understanding
Given the role of warming in albedo change and the projections of increased warming and enhanced melting, future changes in the GrIS albedo will likely result largely from warming and associated feedbacks. We need to quantify and understand these feedbacks so that we can assess the energy budget at the ice sheet surface and predict future changes in ice mass.
References
Benning, L. G., A. M. Anesio, S. Lutz, and M. Tranter (2014), Biological impact on Greenland’s albedo, Nat. Geosci., 7, 691.
Doherty, S. J., T. C. Grenfell, S. Forsström, D. L. Hegg, R. E. Brandt, and S. G. Warren (2013), Observed vertical redistribution of black carbon and other insoluble light-absorbing particles in melting snow, J. Geophys. Res., 118, 5553–5569.
Flanner, M. G., C. S. Zender, J. T. Randerson, and P. J. Rasch (2007), Present-day climate forcing and response from black carbon in snow, J. Geophys. Res., 112, D11202, doi:10.1029/2006JD008003.
Howat, I. M., S. de la Peña, J. H. van Angelen, J. T. M. Lenaerts, and M. R. van den Broeke (2013), Expansion of meltwater lakes on the Greenland ice sheet, Cryosphere, 7, 201–204.
Tedesco, M., J. E. Box, T. S. Jensen, T. Mote, A. K. Rennermalm, L. C. Smith, R. S. W. van de Wal, and J. Wahr (2014), Greenland, Bull. Am. Meteorol. Soc., 95, S136–S138.
van den Broeke, M. R., C. J. P. P. Smeets, and R. S. W. van de Wal (2011), The seasonal cycle and interannual variability of surface energy balance and melt in the ablation zone of the west Greenland ice sheet, Cryosphere, 5, 377–390.
Warren, S. G. (2013), Can black carbon in snow be detected by remote sensing?, J. Geophys. Res., 118, 779–786.
—Marco Tedesco, The City University of New York, New York, N.Y.; email: [email protected]; Sarah Doherty and Stephen Warren, University of Washington, Seattle; Martyn Tranter, University of Bristol, U.K.; Julienne Stroeve, University of Colorado Boulder, Boulder; Xavier Fettweis, University of Liege, Belgium; and Patrick Alexander, The City University of New York, New York, N.Y.
Citation: Tedesco, M., S. Doherty, W. Warren, M. Tranter, J. Stroeve, X. Fettweis, and P. Alexander (2015), What darkens the Greenland ice sheet?, Eos, 96, doi:10.1029/2015EO035773. Published on 17 September 2015.
Text © 2015. The authors. CC BY-NC 3.0
Except where otherwise noted, images are subject to copyright. Any reuse without express permission from the copyright owner is prohibited.