The surface waters of the ocean are heated by the Sun at low latitudes and cooled near the poles. These large-scale patterns of heating and cooling, along with the freezing of sea ice at high latitudes, set up spatial differences in density, driving the so-called thermohaline circulation of the ocean. A major feature of this circulation is the sinking of cold, dense waters at high latitudes. Along the slopes of the Antarctic continent, roughly 25 million cubic meters of water per second are plummeting downward toward the seafloor and then spreading outward, eventually covering the bottom of the entire global ocean and instigating a complex series of related currents that have a major effect on our climate.
Why doesn’t the ocean simply fill up with cold Antarctic water?
A host of mysteries surrounds the details of this process. For example, why doesn’t the ocean simply fill up with this cold Antarctic water? It should only take 1000 years or so, a mere instant on climate timescales, given the rate that water is currently sinking [Munk, 1966]. Scientists now think that turbulence, driven by the breaking of underwater “internal waves,” mixes the heat from warmer surface waters downward and warms the rising waters that originate near the poles. To maintain the oceans in a steady state, where the downward turbulent diffusion of heat balances the upward rise of cold water, roughly 2.6 terawatts (2.6 × 1012 watts) of energy are required [Munk and Wunsch, 1998], roughly equivalent to humanity’s total power consumption.
Turbulence Below
Finding where and how this power is delivered to the deep sea, observing the processes by which undersea waves break, and quantifying the resultant turbulent mixing has proven to be a major scientific challenge. It’s now thought that the tides can supply as much as a third of the needed energy. As surface tides flow across deep-sea topography, they can generate the large propagating internal waves that might significantly mix the ocean when they break.
These internal waves are first cousins to the sea surface waves we’re familiar with. If you take a bucket of water and disturb the surface, gravity will try to restore it back to a flat sheet. This correction “overshoots,” and the disturbance propagates off as surface waves. If the air in the top of the bucket is replaced with a layer of water only slightly less dense than the water below, the same principles apply, except the resulting internal waves move in slow motion. The waves generated as the surface tides flow over undersea topography oscillate once every 12 hours, with heights comparable to the Washington Monument and wavelengths the size of Connecticut.
When the waves eventually cross the ocean basin and come ashore, do they break similar to shoaling surface waves? Is there enough resulting turbulence in the deep sea to affect the balance of the thermohaline circulation?
Picking a Site
To better understand how deep mixing works in the ocean, a team of U.S., Australian, and Canadian scientists sought to study in detail what happens when internal tides hit a continental slope. We sought a clearly defined “beam” of incoming tidal energy that collides with a topographic “target” possessing an interesting range of slopes. (Steeper slopes are expected to reflect the incoming wave, whereas gentle slopes enable the wave to continue to propagate into shallower water. Intermediate, so-called critical slopes lead to wave breaking and deep turbulence.)
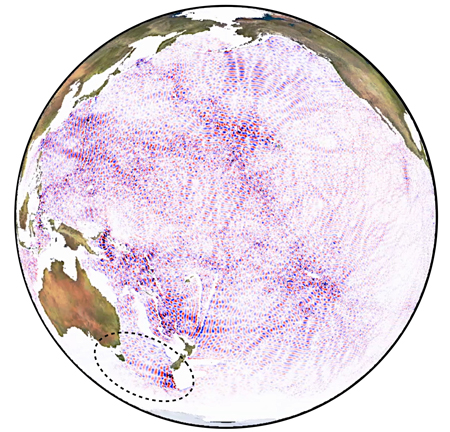
This situation exists in the Tasman Sea (Figure 1). An internal tidal wave with a power density of 4 kilowatts per meter is generated on the Macquarie Ridge south of New Zealand. It travels 1400 kilometers northwest over the course of 4 days and strikes the eastern slopes of Tasmania. What happens next is what we hoped to find out.
We spent the beginning of 2015 in the Southern Ocean deploying sensors and other equipment for the National Science Foundation’s Tasman Tidal Dissipation Experiment (TTIDE). Our primary goal is to figure out what exactly happens when underwater tidal waves impact the eastern continental slopes of Tasmania. With this fundamental information, we then hope to determine the influence of tidal mixing on ocean circulation globally.
TTIDE is guided by a number of recent scientific results, including historic data obtained through the Australian Integrated Marine Observing System, global numerical simulations of the internal tide, satellite measurements of the ocean surface showing transoceanic internal tidal propagation, and measurements of the shoaling deep-sea tide on continental slopes in Virginia, Oregon, and China, where wave reflection as well as dissipation accounts for a significant fraction of the incoming energy.
A Raft of Measurements
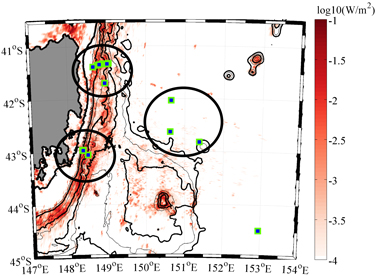
In January 2015, TTIDE scientists launched two undersea gliders and installed 15 deep-sea moorings to initiate the principal field phase of the experiment (Figure 2). The gliders carried instruments that measure electrical conductivity, temperature, and depth (CTD). Together, these sensors provide a picture of fluctuations in seawater density associated with the passing internal tides. From R/V Roger Revelle we deployed two arrays of moorings on the Tasmanian slope: a northern array at a site of suspected high wave energy dissipation and a southern array, where the earlier glider observations suggested that waves reflect strongly off the continental slope. The mooring arrays consisted of high-resolution thermistor chains (Figure 3a) and battery-powered vertical profilers carrying CTDs and ocean current meters. We deployed a third, triangular reflection array offshore to determine the fraction of incident tidal energy that is reflected northeastward back into the Tasman Sea.
Additional measurements were obtained from the Revelle in February and March to further explore the northern and southern sites and to investigate other potential mixing hot spots. These included turbulence measurements, rapid CTD profiling to resolve the internal waves and determine where they broke (Figure 3b), and concurrent density and ocean current profiles from a lowered acoustic Doppler current profiler (LADCP).
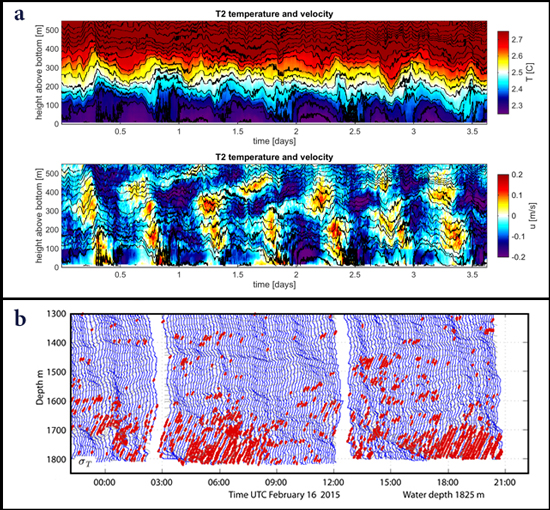
The Pulse of the Oceans
The deep sea, once thought stagnant and quiescent, is, in fact, rather explosive at special sites where the internal tide shoals.
To our delight and relief, as the initial real-time data began to flow into the Revelle’s lab, we saw evidence of giant internal tides breaking 1000–2500 meters down in the sea. Areas of intense turbulence extending 100–200 meters above the sloping seafloor are seen on every tidal cycle. The deep sea, once thought stagnant and quiescent, is, in fact, rather explosive at special sites where the internal tide shoals. Intense turbulent events pulse with a tidal heartbeat.
As the tide breaks, a variety of nonlinear phenomena such as lee waves and bores are found, depending in part on the details of the local topography. These smaller-scale features orchestrate the specific patterns of mixing on the Tasman Slope. As we further explore the TTIDE data, we’ll attempt to uncover the principles governing this complex energy cascade. With this information, we can extend our findings off Tasmania to other sites worldwide to gain a global appreciation of how the tides help to mix the deep sea.
Broadening the Experimental Attack
Extending the TTIDE focus beyond the continental slope, the complementary NSF program T-Beam obtained synoptic measurements (taken simultaneously over a large region) of the incident tidal beam in the central Tasman Sea. T-Beam’s offshore mooring, coupled with extensive LADCP profiling from R/V Falkor, quantified the geometry and energy flux of the beam and documented the rate that internal wave energy attenuates in the open ocean, establishing the initial conditions for TTIDE. The T-Beam investigators isolated the internal tide from the energetic mesoscale (10- to 100-kilometer low-frequency eddies) in the Tasman Sea and confirmed the approximate 4 kilowatt/meter trans-Tasman tidal energy flux.
Concurrently with TTIDE and T-Beam, researchers with the Australian-U.S. program T-Shelf explored the effects of the deep shoaling internal tide in shallow waters up on the Tasmanian continental shelf. The purpose of T-Shelf is to relate the strongly nonlinear phenomena that establish cross-shelf and vertical sediment transport to the interplay of remote and local forces. We deployed two bottom landers, four instrumented moorings, and two Wirewalker wave-powered vertically profiling moorings inshore of the northern array in February, sampling a 15-kilometer section of the outer shelf.
We will use T-Shelf’s shelf break lander data to calculate how much sediment travels across the continental shelf carried by nonlinear internal bores (where the leading edge of the internal wave steepens to become nearly vertical, much like a tidal bore) impinging on the shelf. We believe such resuspension events play an important role in sediment dynamics in coastal waters.
Profiler-mounted microstructure sensors provided continuous, full–water column turbulence observations at two locations on the shelf. We will use these data to establish how the primary energy sources on the shelf, which include the remotely and locally generated internal tides, coastal currents, and local winds, drive turbulent fluxes.
Next Steps
We successfully recovered all moorings by early March, in spite of often-rough seas and energetic mesoscale currents in the region. We are now analyzing the data to determine the fraction of incoming energy that is reflected, document the energetic mesoscale in the region, and quantify the role that evanescent, topographically trapped currents, perhaps indirectly forced by the tides, play in modulating ocean circulation.
The combined TTIDE/T-Beam/T-Shelf experiments will provide a comprehensive view of how the thermohaline balance in the deep ocean is maintained.
As analysis proceeds, the combined TTIDE/T-Beam/T-Shelf experiments will provide a comprehensive view of how deep-sea internal tides propagate and dissipate and how the thermohaline balance in the deep ocean is maintained. This will help scientists better understand ocean circulation and improve the climate models that policy makers rely on.
Acknowledgments
TTIDE and T-Beam were funded by the U.S. National Science Foundation. Participation of the R/V Falkor in T-Beam was provided by the Schmidt Ocean Institute. T-Shelf was supported by grants from the Australian Research Council and The University of Western Australia.
References
Munk, W. (1966), Abyssal recipes, Deep Sea Res., 13, 707–730.
Munk, W., and C. Wunsch (1998), Abyssal recipes II: Energetics of tidal and wind mixing, Deep Sea Res., Part I, 45, 1977–2010.
Author Information
Robert Pinkel, Matthew Alford, Andrew J. Lucas, Shaun Johnston, Jennifer MacKinnon, and Amy Waterhouse, Scripps Institution of Oceanography, San Diego, Calif.; email: [email protected]; Nicole Jones, University of Western Australia, Perth, Australia; Sam Kelly, University of Minnesota, Duluth, Duluth; Jody Klymak, University of Victoria, Victoria, British Columbia, Canada; Jonathan Nash, Oregon State University, Corvallis; Luc Rainville and Zhongxiang Zhao, University of Washington, Seattle; Harper Simmons, University of Alaska Fairbanks; and Peter Strutton, University of Tasmania, Hobart, Australia
Citation: Pinkel, R., et al. (2015), Breaking internal tides keep the ocean in balance, Eos, 96, doi:10.1029/2015EO039555. Published on 17 November 2015.
Correction, 24 November 2015: An earlier version of this article listed authors in a different order and included incorrect affiliations for some authors.
Text © 2015. The authors. CC BY-NC 3.0
Except where otherwise noted, images are subject to copyright. Any reuse without express permission from the copyright owner is prohibited.