When the solar system began to form nearly 4.6 billion years ago, a nebulous cloud of gas and dust surrounded the Sun. One of the gases in that cloud, the noble gas neon, hovered around the region where Earth would soon accrete.
The ratio measurement ruled out that the neon came from some other sources, like meteorites.
Recently, scientists using instruments that can measure isotopes in materials with exacting precision found rocks with neon in them, and they think that it’s the same neon that came from that primordial cloud. This ancient neon found its way into our planet’s interior—or, rather, was pulled there by gravitational forces as the planet formed—the researchers reported in December in Nature.
A discovery like this one, which reveals that neon from the dawn of our solar system resides today in Earth’s interior, was not possible until relatively recently, explained Sujoy Mukhopadhyay, a geochemist at the University of California, Davis, who coauthored the study along with fellow University of California, Davis, geochemist Curtis Williams. Nowadays, continuing improvements in the sensitivity of geochemists’ measurement tools “allow us to make these kinds of isotopic determinations much more precisely than was possible even maybe 10 years ago,” Mukhopadhyay said.
Using a device called a mass spectrometer, or mass spec, that is capable of counting even just a few atoms of a particular isotope in a rock or mineral sample, Williams and Mukhopadhyay determined that the ratio of 20Ne to 22Ne (stable isotopes of neon) in basaltic rocks from mid-ocean volcanic ridges matches that of nebular neon; knowledge of these ratios came from research on isotopes in solar wind samples collected during NASA’s Genesis mission and published in the Astrophysical Journal in 2012. The ratio measurement ruled out that the neon came from some other sources, like meteorites, which have a much lower characteristic 20Ne/22Ne value. “There’s no argument that it could be anything else at this point,” said David Graham, a noble gas geochemist at Oregon State University in Corvallis who was not involved in the study.
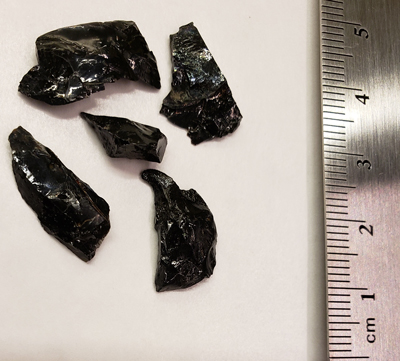
Such is the power of studying isotopes, some of which are unchanging and others of which are radioactive and decay at a fixed rate into other kinds of isotopes. The isotopes 20Ne and 22Ne are stable, and their relative abundances in rocks and other materials may remain steady too. The fixed ratio of their abundances can enable researchers to draw direct lines from elements that exist on Earth today back to the early days of the solar system. On the other hand, thanks to the constant decay rates of radioisotopes, isotopic ratios can also serve as clocks that tell you when a certain event in Earth’s past happened.
The exquisite measurements by Williams and Mukhopadhyay do more than connect our present Earth to when the planet was still in its infancy; they also tell us something about how fast Earth grew up. “If you are going to capture gas, you need a planet that’s big enough” and the gravitational strength that goes with attaining that size, said Graham. Other researchers have shown that the size of Mars is the minimum needed, and “most likely you have to grow bigger than Mars,” said Mukhopadhyay. So the neon ratios indicate that at only a few million years old—and only a few million years after the start of the solar system itself—Earth was big enough to attract and hold onto gases like neon. Moreover, if Earth was able to attract gases like neon, that signifies to scientists that it was also drawing to itself other gases such as water vapor, carbon dioxide, and nitrogen that are vital to life and other important processes and features of the planet. “We are effectively using the neon as a tracer for understanding accretion in the early solar system,” Mukhopadhyay added.
The Lunatics
“You can make these incredibly important statements about nature” by means of isotopic analysis. “You can apply it to almost anything.”
The origins of mass spectrometry and isotopic geochemistry date back to just a few years before AGU was founded a century ago. In work related to his 1897 discovery of the electron, Sir Joseph John “J. J.” Thomson of the University of Cambridge in the United Kingdom devised the first mass spectrometer from 1899 to 1911. After that, he and other researchers developed myriad versions of the instrument and have made startling findings with them ever since. Those findings have included precisely measuring the atomic weights of the elements in the periodic table, verifying Albert Einstein’s formula for the equivalence of energy and mass (E = mc2), and discovering the true age of Earth to be about 4.6 billion years old.
“It seemed like ‘this is magic,’” Don DePaolo recalled thinking when he found out what isotope geochemistry, particularly the study of radioisotopes, was capable of revealing. DePaolo is now at the University of California, Berkeley, but he was conducting research in isotope geochemistry when the field was not so fully formed, in a nebular state of its own. “You can make these incredibly important statements about nature” by means of isotopic analysis, he said. “You can apply it to almost anything.”
In 1973, DePaolo moved to Pasadena, Calif., and started working on his Ph.D. at the California Institute of Technology (Caltech). Back then, the Watergate scandal was still unfolding, the Vietnam War still raged, and, importantly, NASA’s Apollo program had just wrapped up its last mission (Apollo 17) at the end of the prior year. DePaolo accreted onto the radioisotope field at Caltech at the same time that the late geochemist Gerald “Jerry” Wasserburg was busy inventing a new machine that would enable his lab to become the only one in the world that could measure isotopic ratios in the lunar rocks that the Apollo astronauts brought back to Earth.
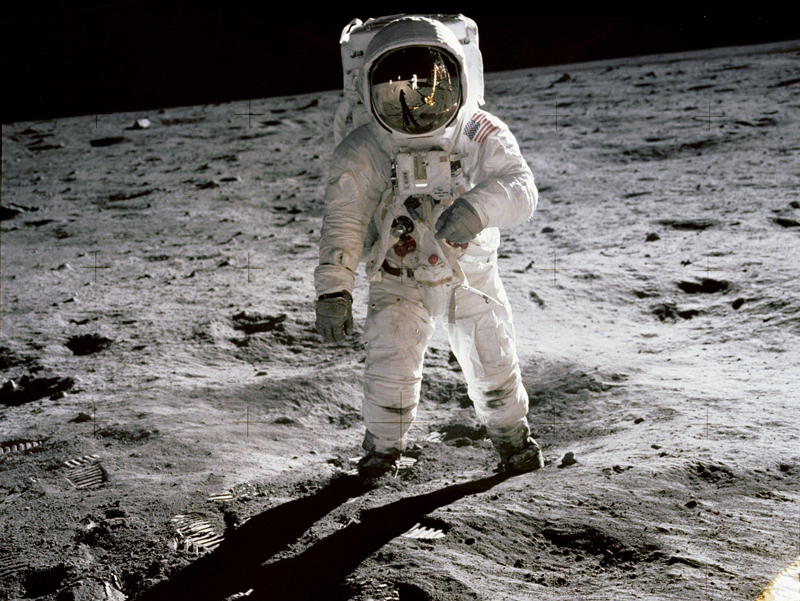
Lunatic I was the first mass spec in the world that could perform digital data collection, the key feature that allowed for the precise measurements needed to analyze the lunar rocks.
The machine was an advanced mass spec. Mass spectrometers count the numbers of atoms of each isotope in a sample of rock or other material by first vaporizing the sample and ionizing its particles. An electric field then propels the ions through a magnetic field that bends the particles’ trajectories in proportion to how heavy they are. In that process, the device separates the isotopes of different masses and measures their relative abundances, or ratios, to one another.
Wasserburg’s breakthrough mass spectrometer came to be known as Lunatic I, and his lab was called the Lunatic Asylum. Lunatic I was the first mass spec in the world that could perform digital data collection, whereas previously, researchers had to measure isotopic abundances on printed sheets of data with a French curve. This improvement, explained Dimitri Papanastassiou, a geochemist and former student of Wasserburg, was the key feature that allowed for the kinds of precise measurements needed to analyze the lunar rocks. Because the Lunatic Asylum was not hampered by what Papanastassiou called the older “analog” methods, he and his colleagues could measure the ratios of isotopes much more precisely, by a factor of about 10, than anyone else.
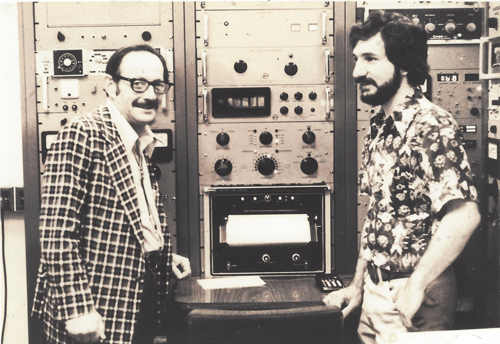
The lunar rocks that the astronauts retrieved do not contain the isotope pairing of potassium and argon that many geochemists used back then to calculate rocks’ ages. They do, however, contain minerals that include small amounts of the radioactive isotope rubidium-87, which started decaying into a stable isotope of strontium at a fixed rate, or half-life, of 48.8 billion years immediately after the lunar minerals formed. Although that tiny but steady rubidium depletion and strontium accumulation provided a way eons later for 1970s scientists to determine the rocks’ ages, “the problem was that you couldn’t date anything with the rubidium-strontium method unless you could measure strontium isotopes extremely precisely,” DePaolo said. “Caltech could do that, in Wasserburg’s lab, and nobody else could do it.”
The lunar rocks came from the darker parts of the Moon, which are ancient basalt flows and cover about 40% of the lunar surface. These rocks, as Wasserburg and Papanastassiou reported in 1970, are between about 3.6 and 3.7 billion years old. “Some people thought the Moon had a very young surface,” Papanastassiou said. But their new age range, he added, solidified the idea that the Moon’s surface is, in fact, extremely old.
Exploring Mantle Mysteries
After illuminating lunar rocks’ ages, which geochemist Bill White of Cornell University in Ithaca, N.Y., described as a “quantum leap” for the field, researchers began applying the methods fashioned by Wasserburg to some puzzles closer to home. For instance, until then the composition of the interior of our own planet was mostly still a mystery; researchers thought that the mantle was entirely made of the same kind of material that had been present in Earth since it first formed, but “we didn’t have a clue,” said DePaolo.
“There’s a huge geochemical cycle working on Earth that extends to through the entire mantle.”
As a window into the mantle, researchers turned to basaltic rocks that had spilled as fiery lavas from volcanoes around the world after rising up as magma from deep within the mantle. It came as a surprise when in the early 1970s a geochemist named Stan Hart found that the isotopic compositions of lead and strontium in basalts that erupted from mid-ocean ridges differed from those produced by volcanoes in places like Hawaii, Tahiti, and Samoa. Hart concluded from his isotopic results that the parts of the mantle from which the lava came must also differ in composition from one another.
White explained that researchers now know that ocean island lavas represent material that was once on the surface of Earth and was subducted down into the deep mantle and then returned via plumes of magma to the surface. After decades of isotopic examination of igneous rocks that have surfaced from many zones of the planet’s innards, the view of the mantle prevailing among geochemists today envisions a vast interior layer almost the opposite of the homogeneous primordial mass that geoscientists had previously hypothesized. Now they think that its contents are a blend of primordial materials that joined the mantle as Earth formed and those that entered it later via the recycling of other rock. “There’s a huge geochemical cycle working on Earth that extends through the entire mantle,” White said.
Settling a Mass Extinction Debate
The quest to understand mass extinctions also often leads to the profound connection between what’s inside our Earth and what transpires at its surface. Take the Permian-Triassic mass extinction, which saw the annihilation of up to 96% of all marine life and, perhaps, about 70% of all land life. Scientists think that the ultimate trigger of the extinction was large-scale volcanism in what is today Siberia, where about 3 million cubic kilometers of lava disgorged onto the planet’s surface over the course of about 1 million years, beginning around 252 million years ago. (That’s enough lava to fill the Mediterranean Sea’s basin about three quarters full.) To draw a connection between the extinction horizon and the volcanism, however, scientists first had to figure out when the Siberian volcanism happened. Enter radioisotopes. By measuring the ratio of uranium and lead isotopes from sedimentary layers, researchers in 2014 determined that the extinction happened between roughly 251,941,000 and 251,880,000 years ago, shortly after the volcanism began.
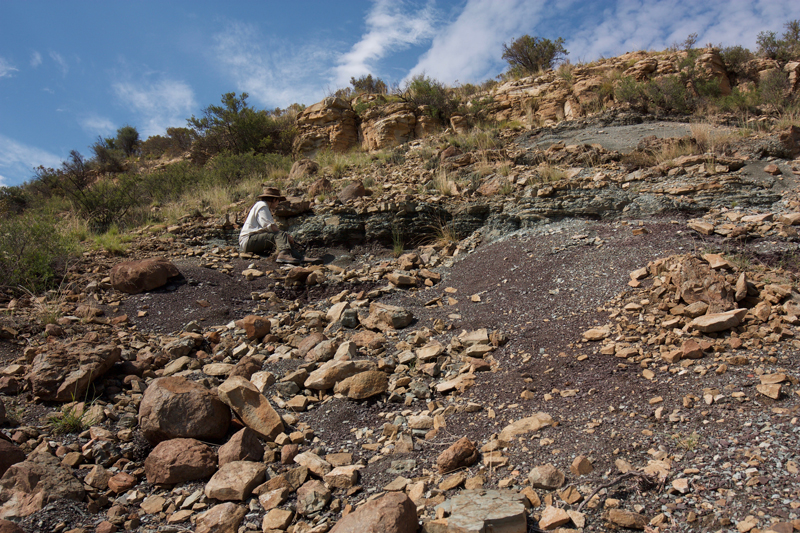
Following improvements in the precision with which isotope geochemists can pinpoint ages of sediments, a radically different picture emerged: The mass extinction of life on land at the Permian-Triassic boundary may have resulted from different causes than the volcanism that extinguished ocean life. That’s because recent high-precision dates of the sedimentary layers close in time to when the land extinction is supposed to have taken place, which were acquired with a mass spec by University of Toronto geochemist Sandra Kamo after she did fieldwork in South Africa, revealed a date of 253.5 million years ago. This finding suggests that the land extinction happened about a million years before the ocean extinction. Having such precise dates, according to DePaolo, is “critical for choosing between different models of what might’ve happened.”
What Makes Supervolcanoes Super?
“One of the things you need to get a supervolcano is a lot of magma—my view is you can’t get that big an eruption unless you have a lot of melting of the Earth’s crust.”
DePaolo, for his part, plans to point his geochemical magnifying glass toward a more modern volcanic mystery: Where does the magma that causes supervolcano eruptions, like the ones that happened in and around what is today Yellowstone National Park in Wyoming, come from? There, an eruption about 2 million years ago coated some 15,000 square kilometers of North America in ash. “The only places where you can form these super eruptions is where the crust is thick,” said DePaolo. “One of the things you need to get a supervolcano is a lot of magma—my view is you can’t get that big an eruption unless you have a lot of melting of the Earth’s crust.”
DePaolo thinks that this is the case because the thicker the crust is, the deeper into the planet the crust goes, and the deeper you go into the planet, the hotter it tends to get. This extra, crustal heating, he believes, is how the vast amounts of magma needed for a supervolcano eruption come to be because a heated, melting crust can provide a near-constant source of magma to a supervolcano. Such is the case in Yellowstone, where the continental crust is relatively thick, and beneath that crust there is a plume of hot mantle material that heats and melts the deepest portions of the crust, which feeds magma into the overlying continent.
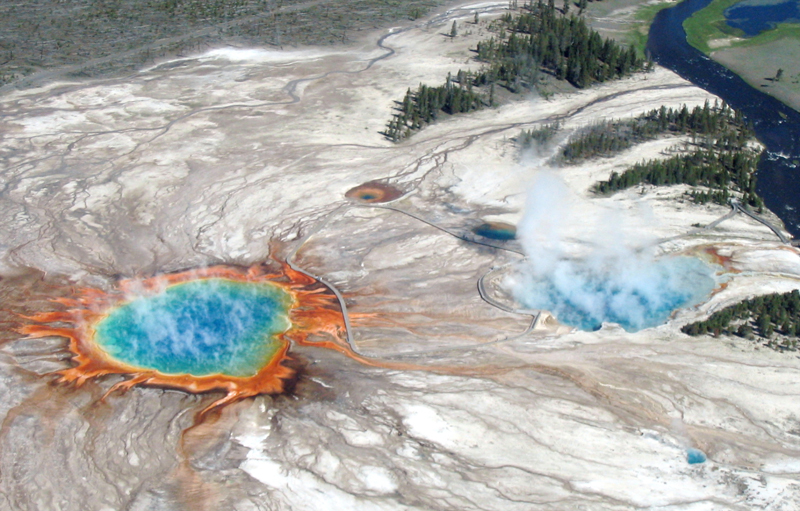
In 2014, University of Utah seismologist Jamie Farrell, along with a team of scientists, published results in Geophysical Research Letters revealing the true size of the magma reservoir that sits just below Yellowstone: It’s about 90 kilometers long and 5 to 17 kilometers deep, which is more than twice as large as researchers thought was the case. Farrell suspects that the presence of such massive reservoirs is what is causing the crust around the supervolcano to be relatively thick. “My take is the thick crust is due to all this material coming up,” he said. But, he added, nobody knows for sure at this point.
“I don’t think people have quite come to the conclusion that it’s as straightforward as I think it is. But we’ll see.”
“This is something that there’s not general agreement on,” DePaolo said. In 1992, he and a team of researchers published a paper in Earth and Environmental Science Transactions of the Royal Society of Edinburgh that highlighted isotopic evidence that he thinks supports the thick crust idea: “The conclusive piece of the puzzle is the neodymium isotope ratios of supervolcano magmas, which indicate that indeed they typically include a lot of melted crustal rock in addition to magma from the mantle,” he said. “I don’t think people have quite come to the conclusion that it’s as straightforward as I think it is. But we’ll see.”
—Lucas Joel ([email protected]), Freelance Journalist
Citation:
Joel, L. (2019), Isotope geochemists glimpse Earth’s impenetrable interior, Eos, 100, https://doi.org/10.1029/2019EO117415. Published on 01 March 2019.
Text © 2019. The authors. CC BY-NC-ND 3.0
Except where otherwise noted, images are subject to copyright. Any reuse without express permission from the copyright owner is prohibited.