In July 2018, our research group set sail for the Calypso hydrothermal vent field in New Zealand’s Bay of Plenty aboard the New Zealand flagship R/V Tangaroa. The voyage was dubbed Quantitative Ocean-Column Imaging (QUOI). Our mission: Find bubbles—lots of them—and record everything they told us about themselves.
The vent field (Figure 1) did not disappoint: It emitted diffuse streams of carbon dioxide and discrete seeps of methane. Our ship’s echo sounders sent out acoustic waves, and we recorded the echoes that these bubbles generated in response. The ways that these echoes varied, depending on the depth of the bubbles and the angles and frequencies of the incoming acoustic waves, provided a wealth of information.
We wondered whether we could use acoustics to fingerprint bubbles in the ocean.
The world’s oceans are full of bubbles. They come from natural sources like dissolving organic matter and gases seeping from great depths below the seafloor and from human sources like leaky oil and natural gas wells and pipelines. Many of these bubbles rise to the surface and release greenhouse gases like carbon dioxide (CO2) and methane (CH4) into the atmosphere.
Some research expeditions have collected preliminary information on bubble streams that they discovered by chance while using echo sounders to map the morphology of the seafloor or search for fish aggregates and even using seismic equipment for subseafloor investigations. Our group wanted to dive deeper into the information that ocean bubbles provide. We wondered whether we could use acoustics to fingerprint bubbles in the ocean.
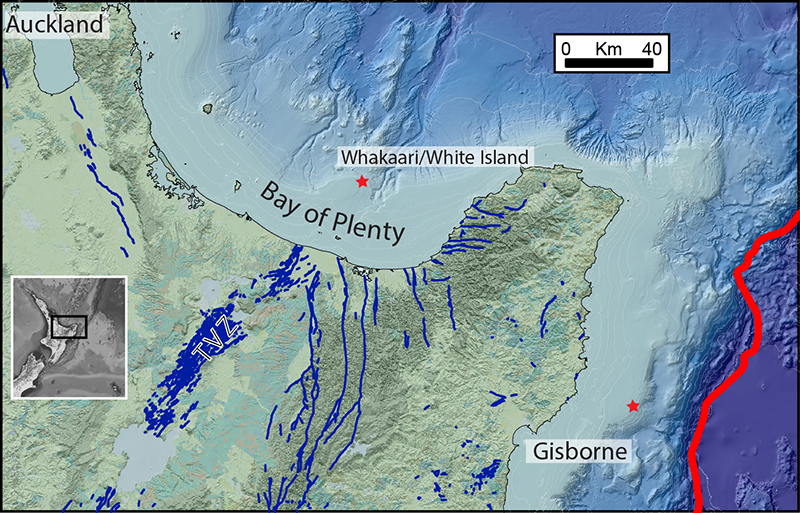
Gathering Clues from Bubbles
Many scientific, industrial, and environmental enterprises make use of the ability to detect, characterize, and quantify bubbles generated from liquid or gaseous features in the ocean, but observations enabling us to measure the volume or identify the types of gases that are released from the seafloor are scarce. Such observations are important as inputs into global carbon cycle models, in helping monitor deep-sea wells and pipelines for potential gas leaks, and in mitigating the associated environmental and economic risks.
Modern echo sounders have been able to image bubble streams in the ocean and trace their movement and behavior as they rise through the water column. To date, analysis of such acoustic data has been largely restricted to localizing and mapping sources of bubbles on the seafloor [e.g., Skarke et al., 2014]. Although there has been some recent progress, it has been more difficult to use echo sounders to quantify and characterize bubble streams (numbers of bubbles, geometry, and composition) and, ultimately, the associated volumetric and mass flow of gas [e.g., Greinert and Nützel, 2004; Weber et al., 2014; Veloso et al., 2015; Weidner et al., 2019].
Differentiating between gas types such as CH4 and CO2, the two most common greenhouse gases released at the seafloor, solely from their acoustic response remains a major challenge. Recent advances in echo sounders, including in our ability to calibrate and use them over a wide range of frequencies and to visualize and analyze their data, have provided a technological arsenal that we can bring to bear on this challenge.
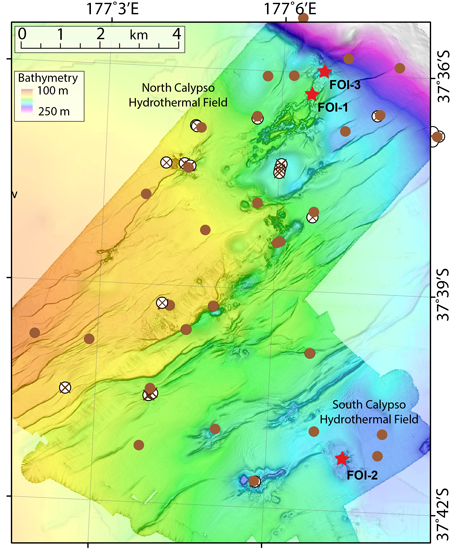
The 3-week QUOI voyage on board R/V Tangaroa used this arsenal to generate a unique data set over the Calypso hydrothermal vent field (Figure 2). The voyage benefited from a 3-year Catalyst: Seeding project, funded by the Royal Society of New Zealand. The project, which launched in April 2017, established a consortium of experts in marine geology, geophysics, and acoustics from seven countries. The data set is now enabling the team to advance the research looking at multidimensional acoustical analysis of the water column, a kind of marine acoustic forensics analysis.
How Do You Characterize a Bubble?
One of the most remarkable of the numerous gas seepages over the New Zealand continental shelf is the Calypso hydrothermal vent field [Stoffers et al., 1999]. Vents emit large bubble streams that can show up on ships’ sonars as gas flares (also called gas plumes). Yet many previous surveys only serendipitously imaged the seeps. These images enable qualitative observations, but gas bubble streams cannot be characterized with conventional oceanographic surveys, which provide only partial physical information on individual bubbles.
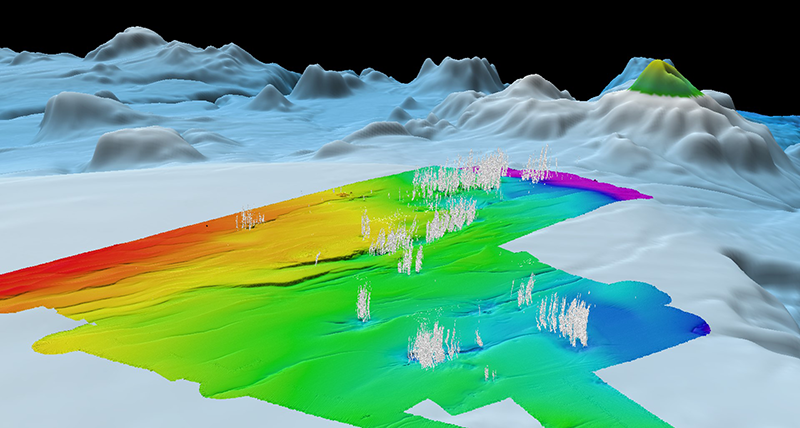
Our project enables us to move beyond locating and defining the shape of the flares to assess the bubble size distribution, differentiate between CH4 and CO2 gases (both of which are known to be released at the Calypso vents), and eventually develop rapid, cost-effective methods of estimating the bubbles’ greenhouse gas flux through the water column.
The intensity of a bubble’s acoustic signal—and whether it generates an echo on a ship’s sonar screen—is related to the bubble’s acoustic scattering cross section, which quantifies the ability of a bubble to reflect the acoustic energy of an incoming acoustic wave back to the echo sounder. This physical (measurable) parameter depends on the geometry, resonance frequency, and damping properties of the bubbles, as well as on the nominal frequency and incident angle of the incoming wave [Clay and Medwin, 1977].
The need to understand how these physical parameters influence acoustic measurements highlights specific requirements for quantitative description of water column gas bubbles. These requirements include calibration of the acoustic devices used to study the bubbles [Ladroit et al., 2018], multifrequency measurements of the backscatter strength of individual bubbles within a plume [Le Gonidec et al., 2002] and how the frequency response of a bubble changes with the ensonification angle (the angle between the sonar beam and the vertical at the point where the beam reaches the bubble), and visual observations for ground truthing. We carried out all of these experiments in 180-meter water depths during the QUOI voyage.
The design of the QUOI voyage included very large overlaps of the sounder seafloor footprints, with up to 95% overlay of adjacent swaths (Figure 3). This enabled us to generate high-resolution images of both the seafloor and the water column, as well as full ensonification of bubbles—immersing them in sound waves with incidence angles from the vertical to a grazing angle of 60°. We were thus able to observe bubble flares from a range of incidence angles. We conducted a robust and complete calibration of all systems at the start of the survey to enable cross correlation between the multiple echo sounder systems. The swath echograms revealed the presence of multiple acoustic flares, and the map of the sum of all echoes within the water column projected on the seafloor highlighted the importance of both the geometry and intensity of the flares and the spatial distribution of the vents on the seafloor (Figure 4).
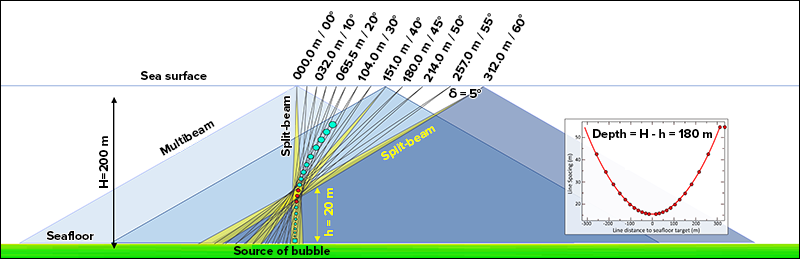
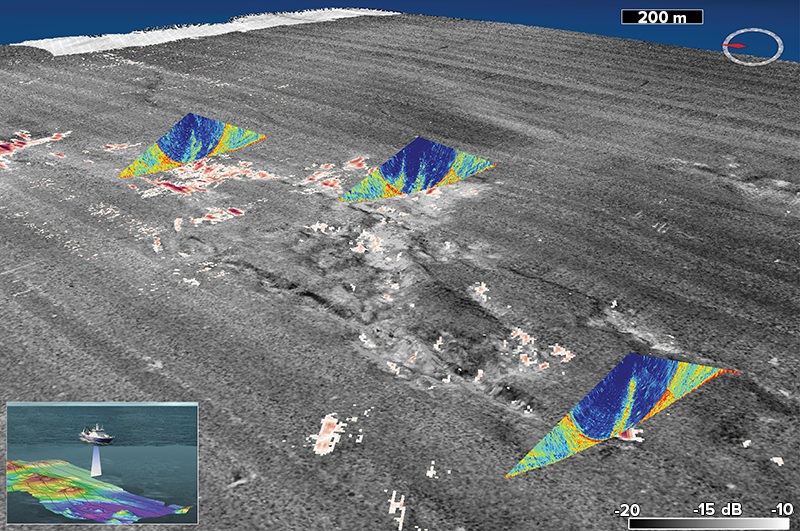
Our Technological Arsenal
Fully characterizing the bubbles required us to explore different echo sounder deployment methods and data acquisition procedures. We used multiple synchronous echo sounders to assess the backscatter strength of targets at different frequencies:
- EM302 and EM2040 multibeam echo sounders collected echo amplitudes athwart and along track simultaneously at 30 and 200 kilohertz.
- An EK60 vertical echo sounder operated simultaneously at 18, 38, 70, 120, and 200 kilohertz.
- A wideband EK80 transducer mounted on a rotatable system emitted pulses in the frequency range of 84–270 kilohertz at different incidence angles.
The voyage deployed three technologically and scientifically innovative systems.
The voyage deployed three technologically and scientifically innovative systems. A mobile unit with a split-beam echo sounder that operates at 38 or 120 kilohertz was deployed directly on the seafloor to ensonify bubble streams horizontally. This generated contrasting images from a different point of view—sideways rather than top down. For 5 days, an autonomous hydrophone placed in the center of a large flare field recorded bubble sounds. We also deployed a synthetic seep generator (or bubble maker) on the seafloor to establish a control data set for many of the bubble parameters. The seep generator creates bubbles with known properties and at known rates and sizes. It allows us to develop an empirical model to which data from natural sites can be compared and to develop a quantitative approach to estimate bubble flux rates.
We collected sediment and water samples for ground truthing of the acoustic signals across the entire Bay of Plenty survey site (Figure 2, brown dots). Video transits allowed us to directly observe most of the active vents identified from high-amplitude echo integration of water column acoustic data (Figure 5, large red spot).
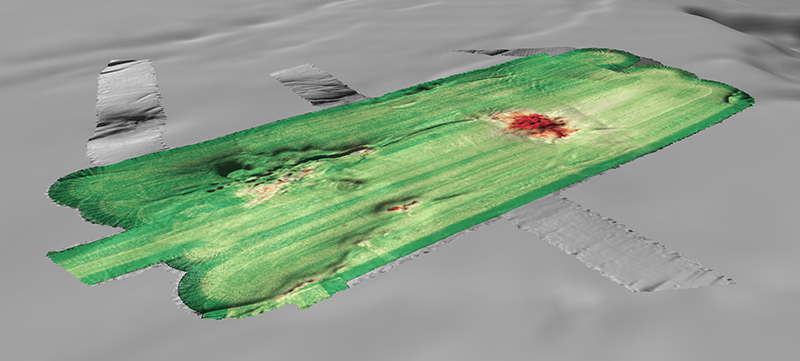
This original, diverse, and multifaceted oceanographic survey has provided us with a data set from which we can develop robust water column surveying methodologies. We anticipate that this will lead to new protocols in identifying, quantifying, and classifying gas bubble streams in the ocean water masses. The QUOI experiments will advance understanding of active geological systems and the relationship the seabed has with the atmosphere.
Acknowledgments
This project is funded by the Royal Society of New Zealand Catalyst: Seeding fund. The QUOI voyage on board R/V Tangaroa was funded by the National Institute of Water and Atmospheric Research. V.L. was supported by the Marine Biodiversity Hub through funding from the Australian government’s National Environmental Science Program.
References
Clay, C. S., and H. Medwin (1977), Acoustical Oceanography: Principles and Applications, 544 + xviii pp., John Wiley, Hoboken, N.J.
Greinert, J., and B. Nützel (2004), Hydroacoustic experiments to establish a method for the determination of methane bubble fluxes at cold seeps, Geo Mar. Lett., 24(2), 75–85, https://doi.org/10.1007/s00367-003-0165-7.
Ladroit, Y., G. Lamarche, and A. Pallentin (2018), Seafloor multibeam backscatter calibration experiment: Comparing 45°-tilted 38-kHz split-beam echosounder and 30-kHz multibeam data, Mar. Geophys. Res., 9(1–2), 41–53, https://doi.org/10.1007/s11001-017-9340-5.
Le Gonidec, Y., D. Gibert, and J.-N. Proust (2002), Multiscale analysis of waves reflected by complex interfaces: Basic principles and experiments, J. Geophys. Res., 107(B9), 2184, https://doi.org/10.1029/2001JB000558.
Skarke, A., et al. (2014), Widespread methane leakage from the sea floor on the northern US Atlantic margin, Nat. Geosci., 7, 657–661, https://doi.org/10.1038/ngeo2232.
Stoffers, P., et al. (1999), Elemental mercury at submarine hydrothermal vents in the Bay of Plenty, Taupo volcanic zone, New Zealand, Geology, 27(10), 931–934, https://doi.org/10.1130/0091-7613(1999)027<0931:EMASHV>2.3.CO;2.
Veloso, M., et al. (2015), A new methodology for quantifying bubble flow rates in deep water using splitbeam echosounders: Examples from the Arctic offshore NW-Svalbard, Limnol. Oceanogr. Methods, 13(6), 267–287, https://doi.org/10.1002/lom3.10024.
Weber, T. C., et al. (2014), Acoustic estimates of methane gas flux from the seabed in a 6000 km2 region in the northern Gulf of Mexico, Geochem. Geophys. Geosyst., 15(5), 1,911–1,925, https://doi.org/10.1002/2014GC00527.
Weidner, E., et al. (2019), A wideband acoustic method for direct assessment of bubble-mediated methane flux, Cont. Shelf Res., 173, 104–115, https://doi.org/10.1016/j.csr.2018.12.005.
Author Information
Geoffroy Lamarche ([email protected]), National Institute of Water and Atmospheric Research (NIWA), Wellington, New Zealand; also at University of Auckland, New Zealand; Yves Le Gonidec, Centre national de la recherche scientifique University of Rennes 1, France; Vanessa Lucieer, Institute for Marine and Antarctic Studies, University of Tasmania, Hobart, Australia; Yoann Ladroit, NIWA, Wellington, New Zealand; Tom Weber, University of New Hampshire, Durham; Arnaud Gaillot, Institut Français de Recherche pour l’Exploration de la Mer (Ifremer), Brest, France; Erin Heffron, University of New Hampshire, Durham; and Sally Watson and Arne Pallentin, NIWA, Wellington, New Zealand
Citation:
Lamarche, G.,Le Gonidec, Y.,Lucieer, V.,Ladroit, Y.,Weber, T.,Gaillot, A.,Heffron, E.,Watson, S., and Pallentin, A. (2019), Gas bubble forensics team surveils the New Zealand ocean, Eos, 100, https://doi.org/10.1029/2019EO133649. Published on 26 September 2019.
Text © 2019. The authors. CC BY-NC-ND 3.0
Except where otherwise noted, images are subject to copyright. Any reuse without express permission from the copyright owner is prohibited.