It’s been more than 300 years since an earthquake with a magnitude greater than 8 has shaken the U.S. Pacific Northwest, but that earthquake was an impressive one. On 26 January 1700, an earthquake with an estimated magnitude of 9 caused the coastline to drop by several feet and a tsunami to inundate the shore.
In the centuries since then, the Juan de Fuca plate has continued to push against the North American plate as it heads downward toward Earth’s mantle, building stress along the Cascadia subduction zone, which extends from northern Vancouver Island in Canada to northern California. As a result, some scientists are estimating as high as a 22% chance of a megathrust earthquake of magnitude 9.0 or greater within the next 50 years [Goldfinger et al., 2017].
The specter of megathrust earthquakes along the Cascadia subduction zone has sparked public interest and prompted widespread hazard preparedness and resilience activities, but nailing down a specific time frame has proven notoriously difficult. One way to overcome such difficulties is to search for evidence of past landslides and compare them with the earthquake record in efforts to tease out the pattern and severity of ground shaking.
Although coastal and offshore geologic evidence provides a long record of regularly occurring earthquakes, data showing whether nearby landslides occurred during or after a given earthquake (or independently of the earthquake) are limited and incomplete. These uncertainties hinder predictions of how much damage future earthquakes are likely to cause.
The Cascadia Earthquake Landslide Working Group, an informal coalition of some 20 scientists with diverse disciplinary backgrounds, recently identified promising means—dating tree rings in forests drowned in landslide-dammed lakes—to establish a regional landslide chronology that we can compare with data sets from past earthquakes. The tree ring work represents one project within the working group, which focuses on a general understanding of landslides in the Cascadia region.
The Past as Uncertain Prologue
Evidence of how Cascadia landscapes fared during past great earthquakes is limited and difficult to acquire.
Although geoscientists have used seafloor and coastal sedimentary archives to produce increasingly refined versions of the Holocene chronology of Cascadia subduction earthquakes [Goldfinger et al., 2012; Witter et al., 2003], they have a poor understanding of what kinds of ground motion and landscape response to expect during future events. Evidence of how Cascadia landscapes fared during past great earthquakes is limited and difficult to acquire, and few data sets exist documenting landslides that have occurred during deep-sourced subduction earthquakes (coseismic response landslides).
The relatively shallow earthquakes along crustal faults produce landslides in a relatively predictable fashion: The larger the event is, the farther its effects tend to radiate into the surrounding terrain. As a result, the cumulative volume of landslide material increases with increasing seismic moment [e.g., Keefer, 1994].
The more powerful 1700 earthquake originated at a much deeper level as the result of one tectonic plate plunging beneath another. Few data inventories exist to document landslides that occur during this type of earthquake (a subduction zone megathrust earthquake), and the few data sets that do exist indicate significant variability in hazard potential.
For example, the 2011 M9.1 Tohoku earthquake that occurred off Japan produced approximately 18 million cubic meters of landslide debris [Wartman et al., 2013]. However, Keefer’s [1994] power law relationship associates this volume of debris with a crustal M6.7 earthquake. The offshore source of this earthquake might explain this dampening of slide activity.
However, this dampening effect was not observed for the M9.5 1960 Chilean earthquake, which caused an estimated 250 square kilometers of ground to fail as landslides [Veblen and Ashton, 1978]. When scaled for volume using the area-volume relationship of Larsen et al. [2010], the Chilean earthquake possibly produced thousands of times more material than the 2011 Tohoku event.
At present, there are no rigorous scientific comparisons of these two events. However, observations of large valley-blocking landslides within glacial sedimentary units in southern Chile [Davis and Karzulovíc K, 1963] suggest that regional lithology (rock characteristics) is an important factor controlling the potential for landslides during an earthquake.
The high variability of documented landslide volumes for past M9 events highlights the need to better understand subduction zone coseismic landslide hazards in the Cascadia subduction zone.
The 1700 Earthquake
To understand how the Cascadia region might respond to quakes in the future, we look to the most recent great earthquake in the region, specifically, the M9 event from 26 January 1700. Although steep, landslide-prone terrain abounds in Cascadia and previously published studies propose tantalizing evidence for earthquake-coupled slope instability [e.g., Schulz et al., 2012], the 1700 earthquake event has not been definitively linked with landslide activity.
Another reason to collect land-based coseismic evidence is to test the hypothesis that megathrust events occur more frequently along the southern segment of the subduction zone [Goldfinger et al., 2012]. If the age of landslide deposits corresponds with Cascadia earthquakes, we can use the pattern and distribution of slope instability to test and calibrate models for megathrust ground motion.
Landslide History Snapshots in Drowned Forests
The Cascadia Earthquake Landslide Working Group used tree ring dating to show, down to the year, when landslide events occurred.
To re-create the landslide history of the Cascadia region, the Cascadia Earthquake Landslide Working Group used dendrochronology (tree ring dating) to show, down to the year, when these events occurred. In fact, the so-called ghost forests of drowned trees in estuaries along the Oregon and Washington coasts provided key evidence for characterizing the precise timing of the 1700 earthquake [Atwater et al., 1991].
The method works like this: Landslides along the steep slopes of the Oregon Coast Range can block stream channels and create small lakes that drown valley forests. The youngest rings on those trees corresponds to the year the trees died, presumably from the drowning. Tree ring widths are strongly correlated with regional climate, and each tree’s unique pattern of ring widths can be compared to a regional chronology established from living trees older than 400 years. Thus, the tree ring signatures for each landslide-damned lake can provide an estimate of when the landslide occurred.
Sediment layers in fore-arc lake basins also record smaller landslides, and they could record a longer record of events [e.g., Morey et al., 2013], albeit with greater age uncertainty. Thanks to the annual resolution of the tree ring records, however, drowned trees record landsliding events down to the year. So if tree ring evidence points to several trees drowning in 1700 near a particular landslide dam, this implies an earthquake origin for the slope failure.
In other words, using drowned trees to link specific landslides to the 1700 earthquake helps to paint a better picture of what exactly happened on the day Earth shook centuries ago. This picture can help scientists better understand the scope of hazards the Northwest faces from such great earthquakes.
Recently, to support one facet of our working group, the U.S. Geological Survey’s National Earthquake Hazards Reduction Program awarded collaborative grants to the University of Oregon, the University of Texas, and the Oregon Department of Geology and Mineral Industries (DOGAMI) to investigate landslide ages from the tree ring record of landslide-dammed lakes (Figure 1). Subsequent mapping and investigation by the team revealed the potential for dendrochronology to establish highly accurate landslide ages based on tree ring chronology [Black et al., 2015].
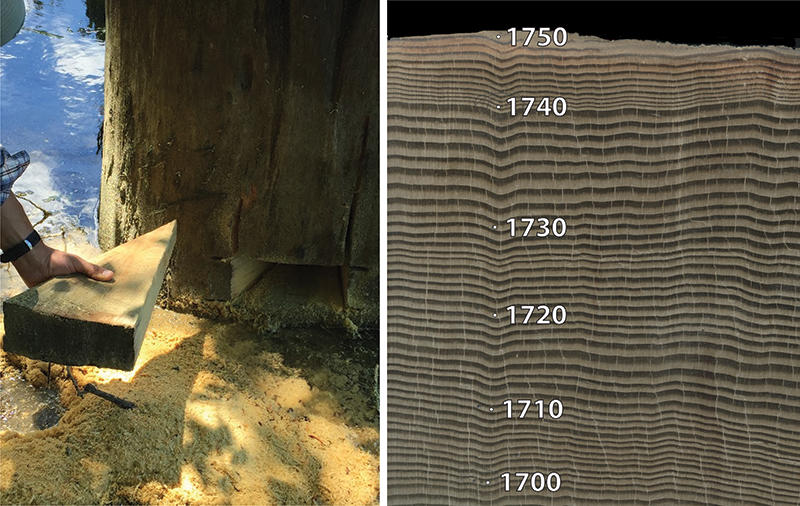
Preliminary results for two landslide-dammed lakes in western Oregon show lake formation dates of 1819 and 1751 [Struble et al., 2017] ( Figure 2). Although these initial findings do not reveal a causal link with the 1700 event, the method holds great promise for establishing formation ages for the more than 200 landslide deposits that impound streams and entomb valley forests in western Cascadia.
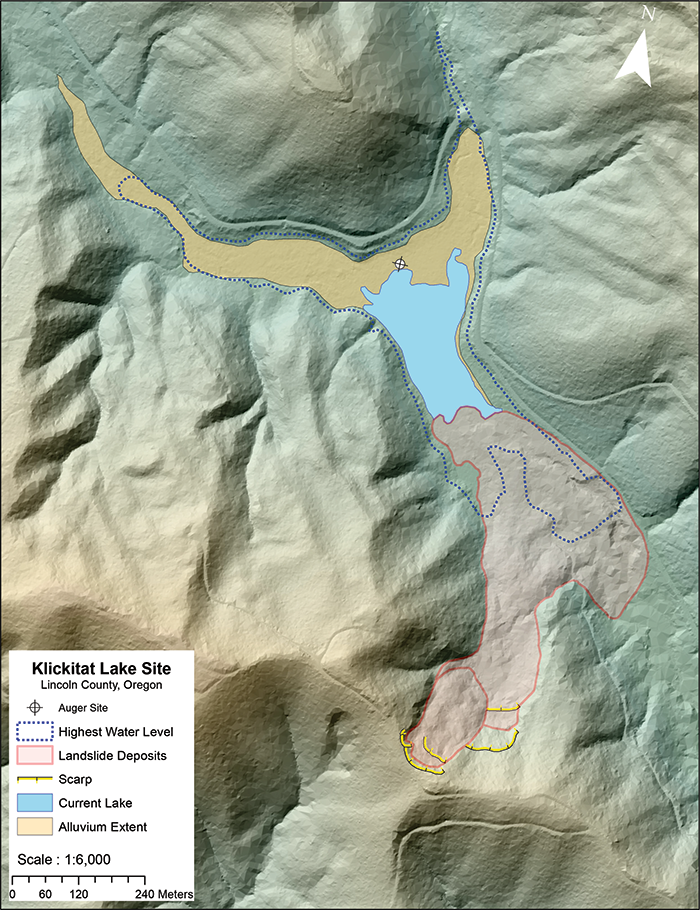
Mapping Landslides Using Topography Data
The working group has identified light detection and ranging (lidar) topographic data as a key asset for landslide mapping in the heavily forested Cascadia terrain [Burns and Madin, 2009]. DOGAMI and the Puget Sound Lidar Consortium have been instrumental in facilitating and serving lidar data (Figure 2), and as a result, Oregon and Washington geoscientists have added thousands of landslides to publicly available landslide inventories.
By combining techniques, including dendrochronologic studies of drowned trees, we may be able to observe the link between landslide ages and Cascadia megathrust events.
Coupling quantitative analysis of landslide roughness with carbon dating of organic material from landslide deposits demonstrates that landslide ages can be estimated through morphologic analysis of surface roughness [Lahusen et al., 2016; Booth et al., 2017]. With these techniques, combined with dendrochronologic studies of drowned trees, we may be able to observe the link between landslide ages and Cascadia megathrust events.
Reading the Historical Record
Through these coordinated efforts, the working group hopes to shed light on the landslide hazards of great Cascadia earthquakes. To spur broader awareness and involvement of the Earth science community, the working group coordinated a technical session at the 2017 Geological Society of America Annual Meeting addressing subduction zone coseismic landslides.
Ultimately, merging regional landslide chronology data sets with new data and ground motion simulations for megathrust earthquakes, such as those produced by the U.S. National Science Foundation–funded M9 Project, should improve our understanding of the connection between subduction zone earthquakes and the response of the terrestrial landscape.
Acknowledgments
Funding for working group efforts was provided by the U.S. Geological Survey Cascadia Recurrence Project. Thoughtful reviews by Karl Wegmann, Sean Gallen, and Scott E. K. Bennett and an anonymous reviewer greatly improved this article.
References
Atwater, B. F., M. Stuiver, and D. K. Yamaguchi (1991), Radiocarbon test of earthquake magnitude at the Cascadia subduction zone, Nature, 353, 156–158, https://doi.org/10.1038/353156a0.
Black, B. A., et al. (2015), Long-term growth-increment chronologies reveal diverse influences of climate forcing on freshwater and forest biota in the Pacific Northwest, Global Change Biol., 21, 594–604, https://doi.org/10.1111/gcb.12756.
Booth, A. M., et al. (2017), Holocene history of deep-seated landsliding in the North Fork Stillaguamish River valley from surface roughness analysis, radiocarbon dating, and numerical landscape evolution modeling, J. Geophys. Res. Earth Surf., 122(2), 456–472, https://doi.org/10.1002/2016JF003934.
Burns, W. J., and I. P. Madin (2009), Protocol for inventory mapping of landslide deposits from light detection and ranging (lidar) imagery, Spec. Pap. 42, 36 pp., Ore. Dep. of Geol. and Mineral Ind., Portland.
Davis, S. N., and J. Karzulovíc K. (1963), Landslides at Lago Riñihue, Chile, Bull. Seismol. Soc. Am., 53, 1,403–1,414.
Goldfinger, C., et al. (2012), Turbidite event history—Methods and implications for Holocene paleoseismicity of the Cascadia subduction zone, U.S. Geol. Surv. Prof. Pap., 1661-F, 170 pp., https://pubs.usgs.gov/pp/pp1661f/.
Goldfinger, C., et al. (2017), The importance of site selection, sediment supply, and hydrodynamics: A case study of submarine paleoseismology on the northern Cascadia margin, Washington USA, Mar. Geol., 384, 4–46, https://doi.org/10.1016/j.margeo.2016.06.008.
Keefer, D. K. (1994), The importance of earthquake-induced landslides to long-term slope erosion and slope-failure hazards in seismically active regions, Geomorphology, 10, 265–284, https://doi.org/10.1016/0169-555X(94)90021-3.
Lahusen, S. R., et al. (2016), Surface roughness dating of long-runout landslides near Oso, Washington (USA), reveals persistent postglacial hillslope instability, Geology, 44(2), 111–114, https://doi.org/10.1130/G37267.1.
Larsen, I. J., D. R. Montgomery, and O. Korup (2010), Landslide erosion controlled by hillslope material, Nat. Geosci., 3(4), 247–251, https://doi.org/10.1038/ngeo776.
Morey, A. E., et al. (2013), Are great Cascadia earthquakes recorded in the sedimentary records from small forearc lakes?, Nat. Hazards Earth Syst. Sci., 13, 2,441–2,463, https://doi.org/10.5194/nhess-13-2441-2013.
Schulz, W. H., S. L. Galloway, and J. D. Higgins (2012), Evidence for earthquake triggering of large landslides in coastal Oregon, USA, Geomorphology, 141–142, 88–98, https://doi.org/10.1016/j.geomorph.2011.12.026.
Struble, W., et al. (2017), Propensity for deep-seated landslides in the Oregon Coastal Ranges during Cascadia megathrust earthquakes through dendrochronological dating of landslide-dammed lakes, Geol. Soc. Am. Abstr. Programs, 49(6), 58-7, https://doi.org/10.1130/abs/2017AM-295761.
Veblen, T. T., and D. H. Ashton (1978), Catastrophic influences on the vegetation of the Valdivian Andes, Chile, Vegetatio, 36, 149–167, https://doi.org/10.1007/BF02342598.
Wartman, J., et al. (2013), Landslides in eastern Honshu induced by the 2011 Tohoku earthquake, Bull. Seismol. Soc. Am., 103(2B), 1,503–1,521, https://doi.org/10.1785/0120120128.
Witter, R. C., H. M. Kelsey, and E. Hemphill-Haley (2003), Great Cascadia earthquakes and tsunamis of the past 6700 years, Coquille River estuary, southern coastal Oregon, Geol. Soc. Am. Bull., 115(10), 1,289–1,306, https://doi.org/10.1130/B25189.1.
Author Information
Jonathan P. Perkins (email: [email protected]), U.S. Geological Survey, Menlo Park, Calif.; Joshua J. Roering, Department of Earth Sciences, University of Oregon, Eugene; William J. Burns, Oregon Department of Geology and Mineral Industries, Portland; Will Struble, Department of Earth Sciences, University of Oregon, Eugene; Bryan A. Black, Marine Science Institute, University of Texas at Austin; Kevin M. Schmidt, U.S. Geological Survey, Menlo Park, Calif.; Alison Duvall, Department of Earth and Space Sciences, University of Washington, Seattle; and Nancy Calhoun, Oregon Department of Geology and Mineral Industries, Portland
Citation:
Perkins, J. P.,Roering, J. J.,Burns, W. J.,Struble, W.,Black, B. A.,Schmidt, K. M.,Duvall, A., and Calhoun, N. (2018), Hunting for landslides from Cascadia’s great earthquakes, Eos, 99, https://doi.org/10.1029/2018EO103689. Published on 08 August 2018.
Text © 2018. The authors. CC BY-NC-ND 3.0
Except where otherwise noted, images are subject to copyright. Any reuse without express permission from the copyright owner is prohibited.