Earth’s hot, pressurized interior is composed primarily of rock that, despite being solid, contains vast stores of fluids rich in carbon and dissolved gases. Unlike the free-flowing waterways of Earth’s surface or the groundwater just beneath, however, these subsurface fluids have long been trapped within minerals deep inside the planet, some since its formation 4.5 billion years ago. Indeed, there is likely more water stored in Earth’s interior than is on its surface, constituting a global ocean in the rock below our feet [Hirschmann, 2006].
We refer to these deep fluids as volatiles—the most common of which include water, carbon dioxide (CO2), and sulfur dioxide (SO2)—because they readily turn to gas in Earth’s near-surface region. However, down below, they often remain chemically bound to minerals or in a liquid state due to the massive pressures they experience. Deep volatiles play critical roles in driving volcanic and plate tectonic processes on our planet. For example, the presence of water in rock reduces the temperatures required to melt rock, which promotes the formation of magmas that feed volcanic eruptions. Deep volatiles can also weaken and damage rocks, allowing Earth to deform more readily by flowing or fracturing, and they influence the occurrence of earthquakes.
Volatiles escape Earth’s interior when they are dissolved in magmas that ascend toward the surface in volcanic regions. As magmas cool and solidify, either as erupted lavas or as igneous rocks emplaced in the crust, they release their dissolved gases, which can then be expelled through fumaroles near volcano summits or through seafloor vents (often called black smokers for the dark billowing plumes of precipitating minerals that pour from them). If, as magma rises to the near surface, the dissolved volatiles transform into gas bubbles that rapidly expand, they can catastrophically fragment magma to drive explosive volcanic eruptions.
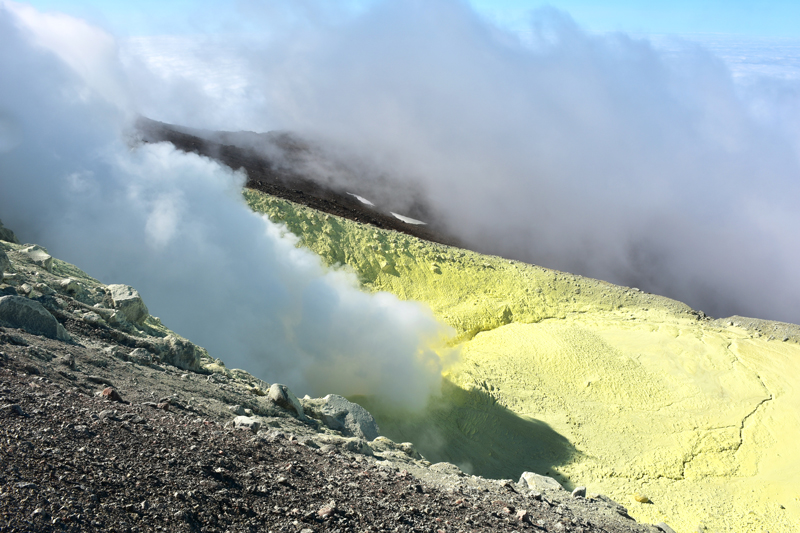
Gases expelled in volcanic regions—water vapor, CO2, SO2, methane, and others—can become incorporated into surface water and into minerals within crustal rocks. They can also, however, collect and reside in our atmosphere for up to thousands of years (although human activities today release much greater amounts of these gases into the atmosphere). The concentrations of these gases in our atmosphere play critical roles in controlling global temperatures.
Understanding how volatiles cycle through our planet is critical for improving our knowledge of Earth’s tectonic and volcanic processes and of the evolution of its climate.
Understanding how volatiles cycle through our planet’s atmosphere, oceans, and rocky interior is thus critical for improving our knowledge of Earth’s tectonic and volcanic processes, as well as of the evolution of its climate both today and through geologic time. Scientific advances in the 20th century provided a foundation for investigations into deep volatile transport during the National Science Foundation–funded MARGINS program (2000–2010) and its successor GeoPRISMS (Geodynamic Processes at Rifting and Subducting Margins) program (2010–2021). Studies occurring within or alongside these programs have since revolutionized our understanding of where volatiles are located, how they are transported from the ocean and the atmosphere into the lithosphere (comprising the crust and the uppermost mantle), and how they are incorporated into magmas on their journey back to Earth’s surface.
Origins of Volcanic Arc Volatiles
The origins of magmatic volatiles remained a controversial topic until relatively recently. Early studies in the 1960s suggested that water dissolved in magma was primarily meteoric, originating as rainfall that circulated within the crust before being incorporated into magma.
Interactions between seawater and rocks at mid-ocean ridges lead to the formation of hydrous minerals that ultimately store components of seawater within oceanic lithosphere.
Beginning in the late 1980s, scientists recognized another, potentially deeper source for volatiles at subduction zones (Figure 1). At these convergent tectonic plate boundaries, where one lithospheric plate sinks beneath another, volatile-laden material is recycled from the shallow and surficial Earth into its deep interior. Most of Earth’s subaerial volcanoes (i.e., those that are exposed to the atmosphere) are found along volcanic arc systems above subduction zones. By examining the abundances of carbon, nitrogen, and noble gases like argon and helium, researchers demonstrated that most of the CO2 and nitrogen released at these volcanoes originated from the subducting plates [Marty and Jambon, 1987]. Later studies showed the same for magmatic water at volcanic arcs [Giggenbach, 1992].
It is now known that the volatiles released at arc volcanoes were originally incorporated into the lithosphere largely through processes occurring within the seafloor millions of years earlier. The global mid-ocean ridge system, a 65,000-kilometer-long divergent plate boundary that accounts for most of Earth’s volcanism, is key in volatile assimilation. These ridges are localities of intense hydrothermal circulation of seawater through the crust, where fluids migrate through developing faults and fractures and fluid convection is driven by heating from submarine volcanism and magmatism. The resulting interactions between seawater and rocks at mid-ocean ridges lead to mineral alteration and the formation of hydrous minerals that ultimately store components of seawater within oceanic lithosphere [ Alt, 1995].
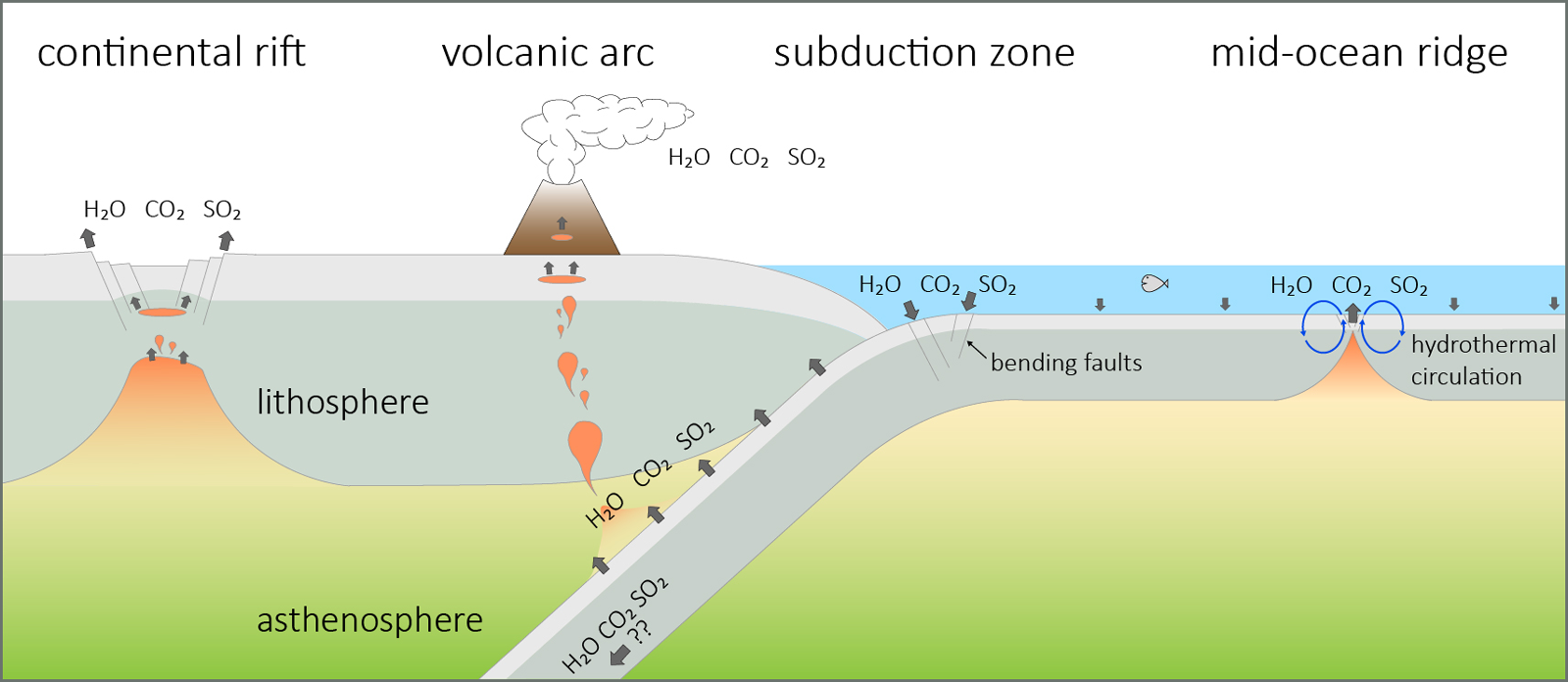
Sediments deposited and rock formed atop seafloor crust as a result of sedimentary processes in ocean basins also store such elements as hydrogen, sulfur, and carbon [ Jarrard, 2003]. And immediately prior to subduction, a final and critical hydration stage results from the bending and faulting of the lithosphere at subduction margins, which enables deeper and more pervasive hydration [Ranero et al., 2003]. Following subduction, some of these volatiles are later released back into the atmosphere as water, CO2, SO2, and other gases because of magmatic and volcanic processes.
Tracking Volatiles Through Subduction Zones
The potential role that faults play in allowing seawater to infiltrate and hydrate oceanic mantle was of wide interest to the MARGINS research community, and investigations of the topic continued during the GeoPRISMS program. Recent work has since confirmed that faults do indeed penetrate the lithosphere, drive fluids into the crust, and likely hydrate the crust and the uppermost mantle [e.g., Grevemeyer et al., 2018]. This work has provided more accurate constraints on the volumetric inputs and outputs of volatiles at subduction zones.
These studies also revealed that although some volatiles delivered into the deep Earth at subduction zones are released back into the atmosphere and the ocean at arc volcanoes, large volumes also remain trapped in the subsurface. Studies now point to higher volatile storage resulting from subduction than previously thought, with these volatiles incorporated into minerals in the crust and the mantle.
Bending-related hydration prior to subduction could contribute the largest amount of water entering subduction zones. Some theoretical and observational studies suggest that volatiles may penetrate tens of kilometers into the oceanic mantle because of this bending [Cai et al., 2018], with the oceanic mantle storing 4 times more water than subducting oceanic crust. However, contrasting studies suggest that volatile penetration is limited to the top few kilometers of oceanic mantle [Korenaga, 2017], leaving the subducting mantle with 4–8 times less water than the overlying oceanic crust. As such, bending-related hydration of the oceanic crust and mantle remains poorly constrained. Refining the large uncertainties in water fluxes at subduction zones is critical for balancing the global water budget.
Missing Sources of Deep Carbon
Because CO2 is a greenhouse gas that plays a key role in controlling Earth’s climate, the scientific community is particularly interested in balancing the outputs and inputs of deep carbon in addition to studying water fluxes. The amount of CO2 in Earth’s atmosphere has remained within a narrow range (typically 150–400 parts per million) throughout the past 10 million or so years, conditions that have maintained an “icehouse climate” (as distinct from “greenhouse climate” periods in the more distant past).
Stable atmospheric CO2 levels require a balance between deep carbon outputs and inputs. But compilations of carbon fluxes reveal that the amount of carbon transported into Earth’s interior by subducting plates significantly exceeds the amount released at volcanic arcs [Kelemen and Manning, 2015]. These observations suggest that the crust and the mantle at subduction zones sequester substantial quantities of carbon, and likely also other critical volatile components.
The apparent deficit in carbon outputs based on current observations and calculations suggests the likelihood of a source of deep carbon.
The apparent deficit in carbon outputs based on current observations and calculations further suggests the likelihood of a currently unrecognized source of deep carbon. Earth’s global mid-ocean ridge system is one possible explanation. At mid-ocean ridges, carbon is extracted from mantle rock when this rock melts and is then released into the ocean during submarine volcanism. Quantifying CO2 fluxes from these settings has been the focus of numerous studies over the past few decades [Wong et al., 2019]. Recently, however, these studies have started to converge on a common value for the total carbon flux from mid-ocean ridges that is too low to make up the apparent carbon deficit, hinting that there are still other critical sources responsible for the remaining carbon.
The GeoPRISMS program targeted studies at other potential sources of deep carbon. In particular, the role of continental rifts (e.g., the East African Rift System) has been of significant interest. Not only are large magma volumes produced at these settings, but they also represent locations where Earth’s continents split apart through the formation of deep cracks and faults (Figure 1).
Recent studies show that in addition to volcanoes, widespread faulting at continental rift systems provides pathways for deep CO2 to reach the surface. Furthermore, compared with mid-ocean ridges, continental rifts occur in comparatively ancient continental lithosphere, thereby tapping volumetrically large carbon stores accumulated over billions of years [Muirhead et al., 2020].
Improving the Picture of Earth’s Volatiles
Over the past few decades, much progress has been made toward constraining global water and carbon fluxes in and out of Earth’s interior. But significant gaps remain in our understanding, particularly of carbon emissions from volcanically and tectonically active regions as well as of fluxes of other important volatiles such as nitrogen, methane, and halogens.
Continental rifts arguably represent the biggest source of uncertainty in global carbon flux estimates.
Continental rifts arguably represent the biggest source of uncertainty in global carbon flux estimates [Plank and Manning, 2019]. Quantifying total CO2 fluxes from continental rifts is thus a critical endeavor for future deep carbon studies. Other uncertain sources of global CO2 outputs, all of which also require further study, include regions of diffuse volatile degassing via soils, volcanic lakes, and volcanic aquifers. However, our ability to measure volatile fluxes at these settings is limited because of their size and complexity. The 3,000-kilometer-long East African Rift System, for example, features thousands of faults. Each fault releases a relatively small amount of gas, but cumulatively they may represent a significant volume of carbon release.
Technological advances using satellite- and unoccupied aerial vehicle–based remote sensing, combined with high-resolution geophysical studies and geodynamic models, may help track emissions from these environments and are needed to obtain a more complete picture of volatile fluxes on Earth. Quantifying these fluxes is relevant for many current and future community scientific efforts and is essential in developing a comprehensive understanding of past and current environmental change on our planet as well as of Earth’s volcanic and seismic hazards.
References
Alt, J. C. (1995), Subseafloor processes in mid-ocean ridge hydrothermal systems, in Seafloor Hydrothermal Systems: Physical, Chemical, Biological, and Geological Interactions, Geophys. Monogr. Ser., vol. 91, edited by S. E. Humphris et al., pp. 85–114, AGU, Washington, D.C.
Cai, C., et al. (2018), Water input into the Mariana subduction zone estimated from ocean-bottom seismic data, Nature, 563, 389–392, https://doi.org/10.1038/s41586-018-0655-4.
Giggenbach, W. F. (1992), Isotopic shifts in waters from geothermal and volcanic systems along convergent plate boundaries and their origin, Earth Planet. Sci. Lett., 113, 495–510, https://doi.org/10.1016/0012-821X(92)90127-H.
Grevemeyer, I., C. R. Ranero, and M. Ivandic (2018), Structure of oceanic crust and serpentinization at subduction trenches, Geosphere, 14, 395–418, https://doi.org/10.1130/GES01537.1.
Hirschmann, M. M. (2006), Water, melting, and the deep Earth H2O cycle, Annu. Rev. Earth Planet. Sci., 34, 629–653, https://doi.org/10.1146/annurev.earth.34.031405.125211.
Jarrard, R. D. (2003), Subduction fluxes of water, carbon dioxide, chlorine, and potassium, Geochem. Geophys. Geosyst., 4(5), 8905, https://doi.org/10.1029/2002GC000392.
Kelemen, P. B., and C. E. Manning (2015), Reevaluating carbon fluxes in subduction zones, what goes down, mostly comes up, Proc. Natl. Acad. Sci. U. S. A., 112, E3997–E4006, https://doi.org/10.1073/pnas.1507889112.
Korenaga, J. (2017), On the extent of mantle hydration caused by plate bending, Earth Planet. Sci. Lett., 457, 1–9, https://doi.org/10.1016/j.epsl.2016.10.011.
Marty, B., and A. Jambon (1987), C3He in volatile fluxes from the solid Earth: Implications for carbon geodynamics, Earth Planet. Sci. Lett., 83, 16–26, https://doi.org/10.1016/0012-821X(87)90047-1.
Muirhead, J. D., et al. (2020), Displaced cratonic mantle concentrates deep carbon during continental rifting, Nature, 582, 67–72, https://doi.org/10.1038/s41586-020-2328-3.
Plank, T., and C. E. Manning (2019), Subducting carbon, Nature, 574, 343–352, https://doi.org/10.1038/s41586-019-1643-z.
Ranero, C. R., et al. (2003), Bending-related faulting and mantle serpentinization at the Middle America Trench, Nature, 425, 367–373, https://doi.org/10.1038/nature01961.
Wong, K., et al. (2019), Deep carbon cycling over the past 200 million years: A review of fluxes in different tectonic settings, Frontiers Earth Sci., 7, 1–22, https://doi.org/10.3389/feart.2019.00263.
Author Information
James D. Muirhead ([email protected]), University of Auckland, Auckland, New Zealand; Samer Naif, Georgia Institute of Technology, Atlanta; Tobias P. Fischer, University of New Mexico, Albuquerque; and Donna J. Shillington, Northern Arizona University, Flagstaff
Citation:
Muirhead, J. D.,Naif, S.,Fischer, T. P., and Shillington, D. J. (2021), Earth’s volatile balancing act, Eos, 102, https://doi.org/10.1029/2021EO155887. Published on 24 March 2021.
Text © 2021. The authors. CC BY-NC-ND 3.0
Except where otherwise noted, images are subject to copyright. Any reuse without express permission from the copyright owner is prohibited.