Centuries of colonization and development have extensively and intensively transformed North American landscapes, particularly in the East, where ecosystems were rapidly converted for agriculture, forestry, mining, and residential use. Colonial towns and cities first appeared along coastlines and rivers before spreading farther inland and west, eventually to be connected by highways, railroads, and power grids. These changes have greatly altered hydrology and accelerated soil erosion across much of the continent, and they have shifted plant and animal communities, introduced contaminants, transformed carbon and nutrient budgets, and altered solute concentrations in water bodies.
Examples of landscape modifications include thinning soils where erosion has outpaced rock weathering and production of new soil and impeded natural replenishment of coastal environments where proliferating dams on streams and rivers interrupt the transport of eroded particles. In short, humans have become major agents of change in shaping the continent’s critical zone—Earth’s near-surface environment that supports terrestrial life—and past legacies and present developments of human action will affect ecosystems and the critical zone for millennia.
For more than a decade, work at sites in the eastern United States has explored the nature and many legacies of human transformation of the critical zone. In the geologically diverse and widely forested hills and valleys of central Pennsylvania, researchers at the Susquehanna Shale Hills Critical Zone Observatory (CZO) have characterized how a legacy of atmospheric pollution continues to shape critical zone processes. In South Carolina, amid the Southern Piedmont, scientists at the Calhoun CZO study effects of historic agriculture that eroded and gullied the region so severely that even 100 years of impressive reforestation has masked more than restored changes in native ecosystems. Meanwhile, in the tall grass prairie of the Midwest, across some of the most fertile soils on Earth, work at the Intensively Managed Landscapes (IML) CZO has focused on understanding how 200 years of industrial agriculture across vast expanses have significantly transformed the landscape inherited from the last ice age.
Here we briefly review discoveries made at these three observatories to show the fundamental importance of human interactions with the critical zone and why further study of these interactions is key to the future of critical zone science.
Critical Zone Contaminants
Throughout the 1800s, forested land within and around the present-day site of the Susquehanna Shale Hills CZO was clear-cut to provide timber for local iron smelters. Although the forests have since regrown, soils there record a history of atmospheric deposition of contaminants from anthropogenic sources, including excess sulfate and nitrate associated with acid rain and elevated levels of metals derived from smelting and coal combustion before 1920 [Brantley et al., 2018].
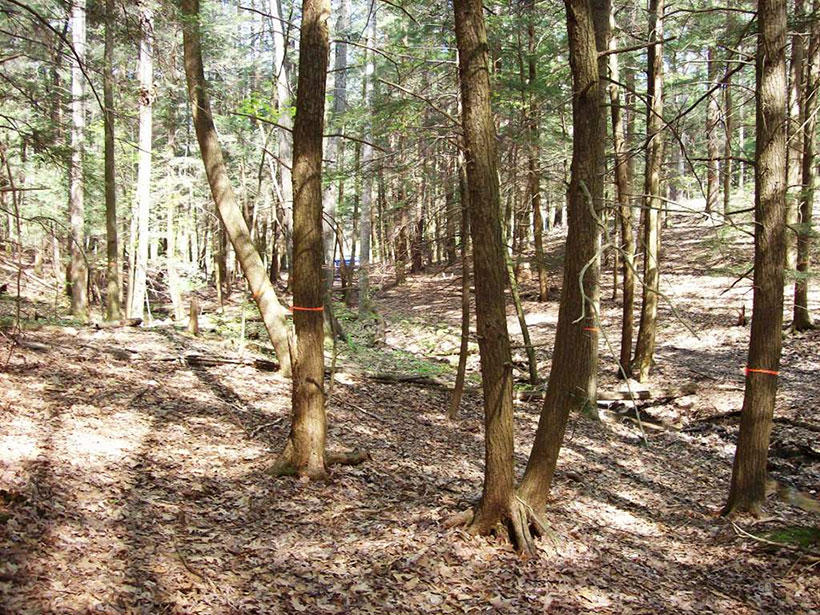
Following deposition, complex interactions within the critical zone have controlled the timescales over which these contaminants persist and are mobilized. For example, despite decades of nitrogen deposition, nitrogen export in streams remains low because of strong retention by forest vegetation and microbial transformations of soil nitrogen to gases like nitrous oxide [Weitzman and Kaye, 2018]. The forest also retains some contaminant metals like manganese, copper, zinc, and cadmium through sequestration in plant biomass and accumulation in decomposing litter [Kraepiel et al., 2015]. In contrast, contaminant lead and molybdenum strongly bind to soil organic matter and are tightly held in surface soils with minimal plant uptake.
Contaminant retention is not uniform across the watershed in the CZO; rather, mobilization and export are strongly controlled by watershed structure. Convergent hillslopes collect and transport runoff and remain at least partially saturated throughout the year, creating transiently low oxygen conditions that favor export of certain contaminants in gaseous and dissolved forms [Weitzman and Kaye, 2018; Brantley et al., 2018].
Understanding critical zone processes, particularly with respect to transport and transformation, is necessary to fully interpret contaminant dynamics.
Accumulation of atmospheric contaminants within watersheds has long-term implications for ecosystem functioning. Nitrogen deposition can relieve nitrogen limitation to organisms while also inducing limitations of other elements like phosphorus. High concentrations of bioavailable metals can also impair tree health: For example, manganese toxicity has been implicated as a driver of sugar maple decline in the region.
The timing and magnitude of contaminant export from watersheds also have implications for aquatic ecosystems. Low retention in soils can lead to pulses of nutrients and metals in streams that drive eutrophication and toxicity, whereas high retention can cause slow release of low but persistent levels of contaminants into aquatic ecosystems. Understanding critical zone processes, particularly with respect to transport and transformation, is necessary to fully interpret these contaminant dynamics.
A Legacy of Erosion
In the mid- to late 1700s, Europeans and enslaved Africans began converting native forests of the Southern Piedmont to agricultural fields [Coughlan and Nelson, 2019]. Initially, fertile bottomlands were cultivated, sometimes on the same fields previously cultivated by Native Americans. As cotton was introduced, immigration to the area and forest clearance accelerated; the degradation represented by soil gullies was reported upon in local newspapers as early as the 1850s. In the post–Civil War era, farms expanded onto steeper hillslopes, and terracing became common for controlling erosion and gully formation [Brecheisen et al., 2019]. The extensive terracing proved ineffective, however, as uplands lost remarkable amounts of soil and bottomlands became inundated with meters-deep legacy sediments [Wade et al., 2020].
By the 1930s, the condition of Piedmont farmlands had degraded to such a degree that farm families extensively abandoned their lands. In the mid-1930s, the U.S. Forest Service (USFS) purchased more than 100,000 hectares of farmland to create Sumter National Forest. The USFS also began to study how to manage and regenerate these fragile, degraded lands by creating the Calhoun Experimental Forest amid what the agency described as “the poorest of Piedmont conditions.” Nearly a century later, the Calhoun CZO was initiated in Sumter National Forest to allow studies of how USFS-promoted reforestation has regenerated the critical zone following the severe disturbances of farming [Richter et al., 2014].
The longer this experiment runs, the more scientists appreciate the legacies of historic agriculture still found in the soil today.
One long-running (and still ongoing) field study quantifying 7 decades of soil change during forest regrowth has become a centerpiece of the work at the Calhoun CZO, revealing, among other findings, how aggrading forests accumulate organic carbon while also depleting soils of nutrient elements. The soils have been greatly acidified during reforestation, which researchers estimate is about 60% attributable to internal ecosystem processes, whereas up to 40% is from acid atmospheric deposition, mainly from sulfur pollution. The longer this experiment runs, the more scientists appreciate the legacies of historic agriculture still found in the soil today. We now understand that past farmers not only accelerated erosional soil loss but also enriched soil fertility with meager amendments of calcium and phosphorus [Richter et al., 2014]. These added nutrients have been recycled by the secondary forests that grow across the landscape today.
Recent critical zone studies based on this long-term experiment have considerably broadened our understanding of consequences on ecosystems from legacy land uses [Mobley et al., 2019]. The picture that has emerged shows that accelerated erosion at the Calhoun site has transformed hillslope morphology and that gully incision drains large volumes of water that otherwise would be available to plants on hillslope soils. The burial of former floodplains with legacy sediments has transformed valley morphology, has filled in bottomlands, and continues to generate elevated sediment loads in downstream waters, a situation that will likely persist for many decades if not many centuries.
Management and Modifications
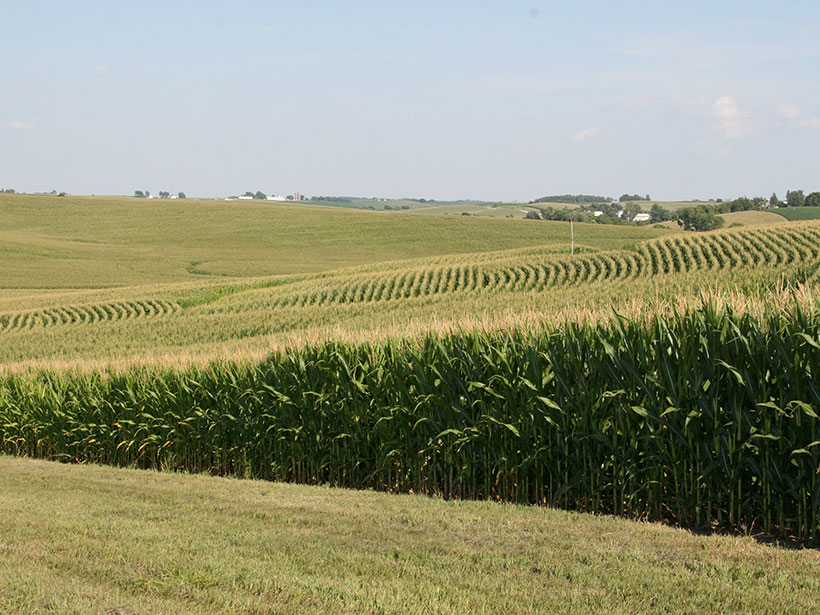
At the IML CZO, which comprises three sites in Illinois, Iowa, and Minnesota, researchers study major human modifications of the critical zone used to achieve high levels of crop production. Land management practices include frequent tillage, widespread fertilizer applications, and subsurface (e.g., tile drains) and surface modifications to improve soil moisture conditions in flat, poorly drained fields [Kumar et al., 2018]. These active and energy-intensive anthropogenic modifications enhance crop production, but they also promote erosion of soil and soil organic carbon from uplands and their deposition in bottomlands, changes in the physical and chemical characteristics of soil [Yan et al., 2019], and increased nutrient and sediment transport into streams.
These processes degrade soil and water quality both locally and far downstream. Many studies, for example, demonstrate how the transport of excess nutrients, primarily from Midwestern farmland, into the Gulf of Mexico causes serious eutrophication and hypoxia that result in toxic conditions for marine life [e.g., Khanna et al., 2019]. IML CZO studies have led to improved understanding of how critical zone processes respond to prevailing industrial agriculture, helping guide sustainable production that uses model- and data-driven precision nutrient management [Khanna et al., 2019].
With measurements and modeling made possible by in situ data and high-resolution remote sensing techniques, new insights have emerged into how physical and chemical alterations of the critical zone interact to control nutrient dynamics in intensive agriculture.
With measurements and modeling made possible by in situ data and high-resolution remote sensing techniques such as lidar and hyperspectral imaging, new insights have emerged into how physical and chemical alterations of the critical zone interact to control nutrient dynamics in intensive agriculture. Intensive land management modifies the microtopography and roughness of the land surface, altering conductivity, pore structure, and preferential pathways for flow and runoff in the critical zone. These alterations then modify soil structure and strength, erosion, soil organic carbon transport and distribution, moisture persistence, and associated geochemical properties.
For example, the ages and concentrations of nutrients distributed across the landscape depend on fertilizer applications as well as on subsequent nutrient mobilization and transformation, which are influenced by topography and soil variability and by water flows affected by stream channelization and subsurface tile drains. Although soluble nitrate moves quickly through tile drains during rainstorms, ammonium accumulates in the soil and contributes to long-term legacy nitrogen impacts. Episodic ponding in topographic depressions and spacing of drainage lines in tile drains add substantial spatial variability in nutrient distribution, with low concentrations of older nitrogen amassed away from tile drains and high concentrations of younger nitrogen found near tile drains.
The age-concentration dynamics of nutrients above and below subsurface tile drain networks are also very different because nitrogen that passes below the drain lines moves much slower through the soil [Woo and Kumar, 2019]. Agricultural crops also regulate nutrient dynamics by enhancing or inhibiting microbial activity in the root zone, which, along with soil chemistry, influences nutrient concentrations reaching nearby waterways [Roque-Malo et al., 2020]. Such findings have helped elucidate the complex interactions and downstream impacts resulting from industrial agriculture practices.
A Deeply Coupled System
Eastern North America, like much of the world, features an extensive mosaic of ecosystems challenged by modern and legacy impacts that have altered critical zone processes with centurial and millennial memories. Physical modifications combined with direct and indirect chemical inputs are resetting rates of geochemical transformation and transport such that, compared with areas where human-induced landscape modification has been minimal, many altered landscapes now have reduced capacities to process excess nutrients and contaminants before transmitting them to other ecosystems. Where erosion is significant, the structure and function of the critical zone may be irrevocably altered.
Humans have, indeed, become a major agent in changing Earth’s critical zone. Critical zone research is helping to reveal not only how we are reshaping the landscape but also how complex feedbacks between critical zone components lead to legacies of degraded terrestrial ecosystems and their waters. To continue advancing this understanding of human-natural interactions, future critical zone science must increasingly focus on the critical zone as a deeply coupled and novel human-natural system.
References
Brantley, S. L., et al. (2018), Susquehanna Shale Hills critical zone observatory: Shale Hills in the context of Shaver’s Creek Watershed, Vadose Zone J., 17(1), 1–19, https://doi.org/10.2136/vzj2018.04.0092.
Brecheisen, Z. S., et al. (2019), Micro-topographic roughness analysis (MTRA) highlights minimally eroded terrain in a landscape severely impacted by historic agriculture, Remote Sens. Environ., 222, 78–89, https://doi.org/10.1016/j.rse.2018.12.025.
Coughlan, M. R., and D. R. Nelson (2019), Geostatistical analysis of historical contingency and land use footprints in the prehistoric settlement dynamics of the South Carolina Piedmont, North America, J. Archaeol. Sci., 107, 1–9, https://doi.org/10.1016/j.jas.2019.04.003.
Khanna, M., et al. (2019), Harnessing emerging technologies to address the hypoxia challenge, Nat. Sustainability, 2, 889–891, https://doi.org/10.1038/s41893-019-0381-4.
Kraepiel, A. M. L., et al. (2015), Natural and anthropogenic processes contributing to metal enrichment in surface soils of central Pennsylvania, Biogeochemistry, 123(1–2), 265–283, https://doi.org/10.1007/s10533-015-0068-5.
Kumar, P., et al. (2018), Critical transition in critical zone of intensively managed landscapes, Anthropocene, 22, 10–19, https://doi.org/10.1016/j.ancene.2018.04.002.
Mobley, M. L., et al. (2019), How to estimate statistically detectable trends in a time series: A study of soil carbon and nutrient concentrations at the Calhoun LTSE, Soil Sci. Soc. Am. J., 83, S133–S140, https://doi.org/10.2136/sssaj2018.09.0335.
Richter, D. D., et al. (2014), Evolution of soil, ecosystem, and critical zone research at the USDA FS Calhoun Experimental Forest, in USDA Forest Service Experimental Forests and Ranges, pp. 405–433, Springer, New York, https://doi.org/10.1007/978-1-4614-1818-4_18.
Roque-Malo, S., D. K. Woo, and P. Kumar (2020), Modeling the role of root exudation in critical zone nutrient dynamics, Water Resour. Res., 56, e2019WR026606, https://doi.org/10.1029/2019WR026606.
Wade, A. M., et al. (2020), Limited carbon contents of centuries old soils forming in legacy sediment, Geomorphology, 354, 107018, https://doi.org/10.1016/j.geomorph.2019.107018.
Weitzman, J. N., and J. P. Kaye (2018), Nitrogen budget and topographic controls on nitrous oxide in a shale‐based watershed, J. Geophys. Res. Biogeosci., 123(6), 1,888–1,908, https://doi.org/10.1029/2017JG004344.
Woo, D. K., and P. Kumar, (2019), Impacts of subsurface tile drainage on age-concentration dynamics of inorganic nitrogen in soil, Water Resour. Res., 55, 1,470–1,489, https://doi.org/10.1029/2018WR024139.
Yan, Q., et al. (2019), Three-dimensional modeling of the coevolution of landscape and soil organic carbon, Water Resour. Res., 55, 1,218–1,241, https://doi.org/10.1029/2018WR023634.
Author Information
Praveen Kumar ([email protected]), University of Illinois at Urbana-Champaign, Urbana; Elizabeth Herndon, Oak Ridge National Laboratory, Oak Ridge, Tenn.; and Daniel D. Richter, Duke University, Durham, N.C.
Citation:
Kumar, P.,Herndon, E., and Richter, D. D. (2020), Critical agents of change at Earth’s surface, Eos, 101, https://doi.org/10.1029/2020EO149750. Published on 29 September 2020.
Text © 2020. The authors. CC BY-NC-ND 3.0
Except where otherwise noted, images are subject to copyright. Any reuse without express permission from the copyright owner is prohibited.