The popular media occasionally warns of an impending earthquake—the “big one”—that could devastate the U.S. Pacific Northwest and coastal British Columbia, Canada. Although ranging into hyperbole at times, such shocking coverage provides wake-up calls that the region is indeed vulnerable to major earthquake hazards courtesy of the Cascadia Subduction Zone (CSZ).
The last behemoth earthquake on the Cascadia Subduction Zone (CSZ) struck on 26 January 1700. We know this age because of several lines of geologic proxy evidence, in addition to Japanese historical records.
The CSZ is a tectonic boundary off the coast that has unleashed massive earthquakes and tsunamis as the Juan de Fuca Plate is thrust beneath the North American Plate. And it will do so again. But when? And how big will the earthquake—or earthquakes—be?
The last behemoth earthquake on the CSZ, estimated at magnitude 9, struck on 26 January 1700. We know this age with such precision—unique in paleoseismology—because of several lines of geologic proxy evidence that coalesce around that date, in addition to Japanese historical records describing an “orphan tsunami” (a tsunami with no corresponding local earthquake) on that particular date [Atwater et al., 2015]. Indigenous North American oral histories also describe the event. Geoscientists have robust evidence for other large earthquakes in Cascadia’s past; however, deciphering and precisely dating the geologic record become more difficult the farther back in time you go.
Precision dating of and magnitude constraints on past earthquakes are critically important for assessing modern CSZ earthquake hazards. Such estimates require knowledge of the area over which the fault has broken in the past; the amount of displacement, or slip, on the fault; the speed at which slip occurred; and the timing of events and their potential to occur in rapid succession (called clustering). The paucity of recent seismicity on the CSZ means our understanding of earthquake recurrence there primarily comes from geologic earthquake proxies, including evidence of coseismic land level changes, tsunami inundations, and strong shaking found in onshore and marine environments (Figure 1). Barring modern earthquakes, increasing the accuracy and precision of paleoseismological records is the only way to better constrain the size and frequency of megathrust ruptures and to improve our understanding of natural variability in CSZ earthquake hazards.
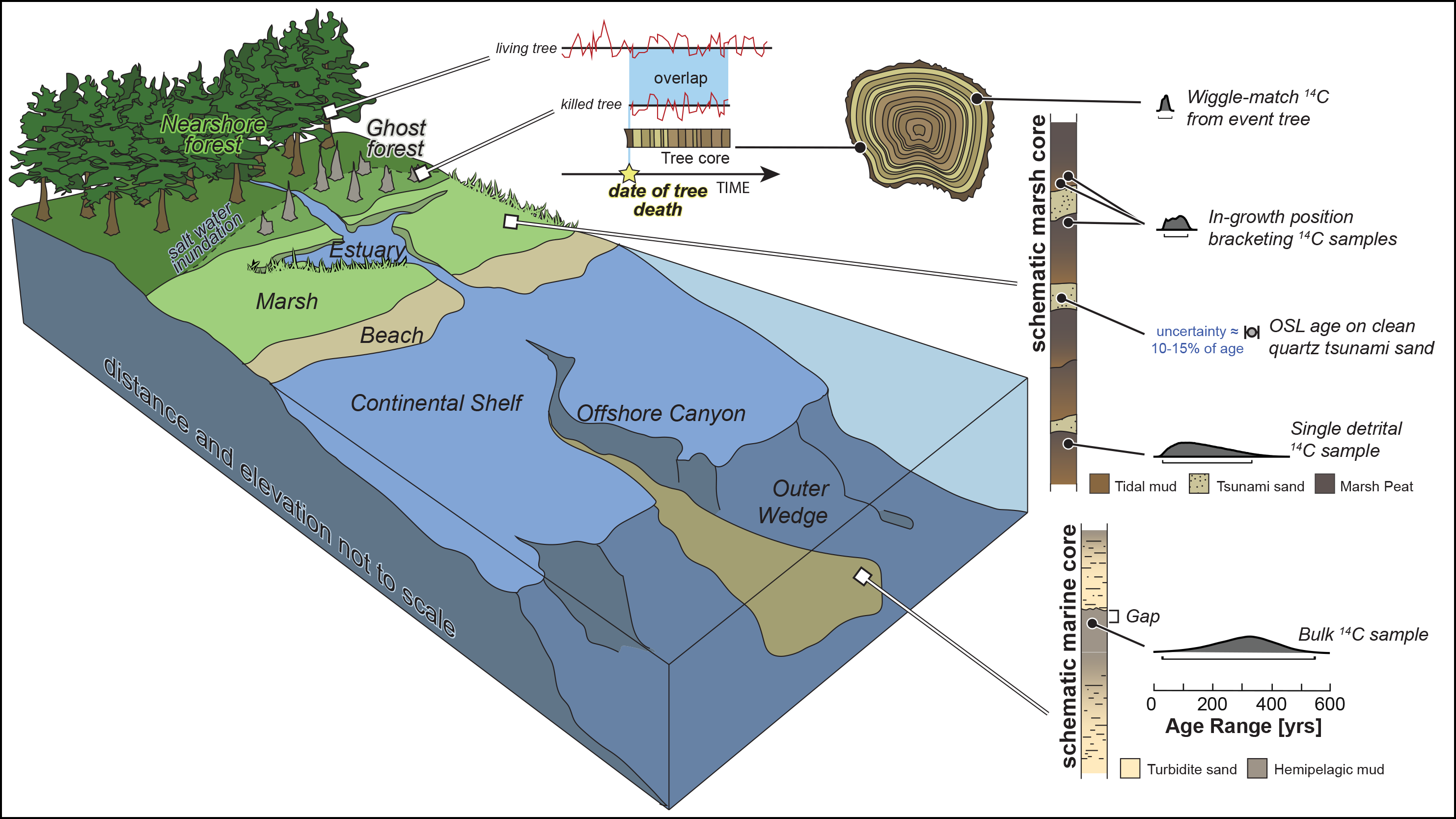
To discuss ideas, frontiers, and the latest research at the intersection of subduction zone science and geochronology, a variety of specialists attended a virtual workshop about earthquake recurrence on the CSZ hosted by the U.S. Geological Survey (USGS) in February 2021. The workshop, which we discuss below, was part of a series that USGS is holding as the agency works on the next update of the National Seismic Hazard Model, due out in 2023.
Paleoseismology Proxies
Cascadia has one of the longest and most spatially complete geologic records of subduction zone earthquakes, stretching back more than 10,000 years along much of the 1,300-kilometer-long margin, yet debate persists over the size and recurrence of great earthquakes [Goldfinger et al., 2012; Atwater et al., 2014]. The uncertainty arises in part because we lack firsthand observations of Cascadia earthquakes. Thus, integrating onshore and offshore proxy records and understanding how different geologic environments record past megathrust ruptures remain important lines of inquiry, as well as major hurdles, in CSZ science. These hurdles are exacerbated by geochronologic data sets that differ in their precision and usefulness in revealing past rupture patches.
One of the most important things to determine is whether proxy evidence records the CSZ rupturing in individual great events (approximately magnitude 9) or in several smaller, clustered earthquakes (approximately magnitude 8) that occur in succession. A magnitude 9 earthquake releases 30 times the energy of a magnitude 8 event, so the consequences of misinterpreting the available data can result in substantial misunderstanding of the seismic hazard.
Some of the best proxy records for CSZ earthquakes lie onshore in coastal environments, such as coastal wetlands.
Geologic proxies of megathrust earthquakes are generated by different aspects of the rupture process and can therefore inform us about specific rupture characteristics and hazards. Some of the best proxy records for CSZ earthquakes lie onshore in coastal environments. Coastal wetlands, for example, record sudden and lasting land-level changes in their stratigraphy and paleoecology when earthquakes cause the wetlands’ surfaces to drop into the tidal range (Figure 1) [Atwater et al., 2015]. The amount of elevation change that occurs during a quake, called “coseismic deformation,” can vary along the coast during a single event because of changes in the magnitude, extent, and style of slip along the fault [e.g., Wirth and Frankel, 2019]. Thus, such records can reveal consistency or heterogeneity in slip during past earthquakes.
Tsunami deposits onshore are also important proxies for understanding coseismic slip distribution. Tsunamis are generated by sudden seafloor deformation and are typically indicative of shallow slip, near the subduction zone trench (Figure 1) [Melgar et al., 2016]. The inland extent of tsunami deposits, and their distribution north and south along the subduction zone, can be used to identify places where an earthquake caused a lot of seafloor deformation and can tell generally how much displacement was required to create the tsunami wave.
Offshore, seafloor sediment cores show coarse layers of debris flows called turbidites that can also serve as great proxies for earthquake timing and ground motion characteristics. Coseismic turbidites result when earthquake shaking causes unstable, steep, submarine canyon walls to fail, creating coarse, turbulent sediment flows. These flows eventually settle on the ocean floor and are dated using radiocarbon measurements of detrital organic-rich material.
Geochronologic Investigations
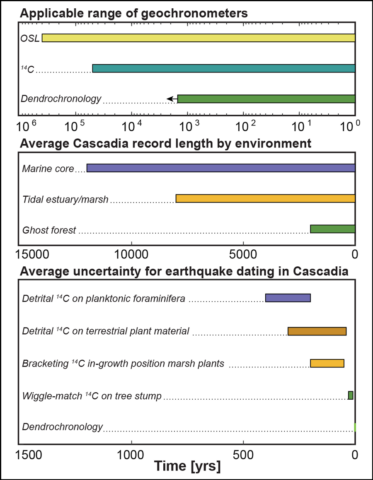
To be useful, proxies must be datable. Scientists primarily use radiocarbon dating to put past earthquakes into temporal context. Correlations in onshore and offshore data sets have been used to infer the occurrence of up to 20 approximately magnitude 9 earthquakes on the CSZ over the past 11,000 years [Goldfinger et al., 2012], although uncertainty in the ages of these events ranges from tens to hundreds of years (Figure 2). These large age uncertainties allow for varying interpretations of the geologic record: Multiple magnitude 8 or magnitude 7 earthquakes that occur over a short period of time (years to decades) could be misidentified as a single huge earthquake. It’s even possible that the most thoroughly examined CSZ earthquake, in 1700, might have comprised a series of smaller earthquakes, not one magnitude 9 event, because the geologic evidence providing precise ages of this event comes from a relatively short stretch of the Cascadia margin [Melgar, 2021].
By far, the best geochronologic age constraints for CSZ earthquakes come from tree ring, or dendrochronological, analyses of well-preserved wood samples [e.g., Yamaguchi et al., 1997], which can provide annual and even seasonal precision (Figure 2). Part of how scientists arrived at the 26 January date for the 1700 quake was by using dendrochronological dating of coastal forests in southwestern Washington that were killed rapidly by coseismic saltwater submergence. Some of the dead western red cedar trees in these “ghost forests” are preserved with their bark intact; thus, they record the last year of their growth. By cross dating the dead trees’ annual growth rings with those in a multicentennial reference chronology derived from nearby living trees, it is evident that the trees died after the 1699 growing season.
The ghost forest, however, confirms only that coseismic submergence in 1700 occurred along the 90 kilometers of the roughly 1,300-kilometer-long Cascadia margin where these western red cedars are found. The trees alone do not confirm that the entire CSZ fault ruptured in a single big one.
Meanwhile, older CSZ events have not been dated with such high accuracy, in part because coseismically killed trees are not ubiquitously distributed and well preserved along the coastline and because there are no millennial-length, species-specific reference chronologies with which to cross date older preserved trees (Figure 2).
Advances in Dating
At the Cascadia Recurrence Workshop earlier this year, researchers presented recent advances and discussed future directions in paleoseismic dating methods. For example, by taking annual radiocarbon measurements from trees killed during coseismic coastal deformation, we can detect dated global atmospheric radiocarbon excursions in these trees, such as the substantial jump in atmospheric radiocarbon between the years 774 and 775 [Miyake et al., 2012]. This method allows us to correlate precise dates from other ghost forests along the Cascadian coast from the time of the 1700 event and to date past megathrust earthquakes older than the 1700 quake without needing millennial-scale reference chronologies [e.g., Pearl et al., 2020]. Such reference chronologies, which were previously required for annual age precision, are time- and labor-intensive to develop. With this method, new data collections from coastal forests that perished in, or survived through, CSZ earthquakes can now give near-annual dates for both inundations and ecosystem transitions.
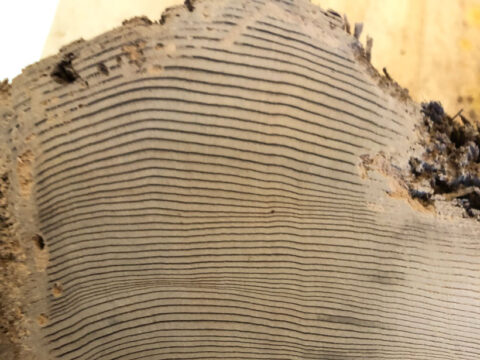
Although there are many opportunities to pursue with dendrochronology, such as dating trees at previously unstudied sites and trees killed by older events, we must supplement this approach with other novel geochronological methods to fill critical data gaps where trees are not preserved. Careful sampling and interpretation of age results from radiocarbon-dated material other than trees can also provide tight age constraints for tsunami and coastal submergence events.
For example, researchers collected soil horizons below (predating) and overlying (postdating) a tsunami deposit in Discovery Bay, Wash., and then radiocarbon dated leaf bases of Triglochin maritima, a type of arrowgrass that grows in brackish and freshwater marsh environments. The tsunami deposits, bracketed by well-dated pretsunami and posttsunami soil horizons, revealed a tsunamigenic CSZ rupture that occurred about 600 years ago on the northern CSZ, perhaps offshore Washington State and Vancouver Island [Garrison-Laney and Miller, 2017].
Multiple bracketing ages can dramatically reduce uncertainty that plagues most other dated horizons, especially those whose ages are based on single dates from detrital organic material (Figure 2). Although the age uncertainty of the 600-year-old earthquake from horizons at Discovery Bay is still on the order of several decades, the improved precision is enough to conclusively distinguish the event from other earthquakes dated along the margin.
Further advancements in radiocarbon dating continue to provide important updates for dating coseismic evidence from offshore records. Marine turbidites do not often contain materials that provide accurate age estimates, but they are a critically important paleoseismic proxy [Howarth et al., 2021]. Turbidite radiocarbon ages rely on correcting for both global and local marine reservoir ages, which are caused by the radiocarbon “memory” of seawater. Global marine reservoir age corrections are systematically updated by experts as we learn more about past climates and their influences on the global marine radiocarbon reservoir [Heaton et al., 2020]. However, samples used to calibrate the local marine reservoir corrections in the Pacific Northwest, which apply only to nearby sites, are unfortunately not well distributed along the CSZ coastline, and little is known about temporal variations in the local correction, leading to larger uncertainty in event ages.
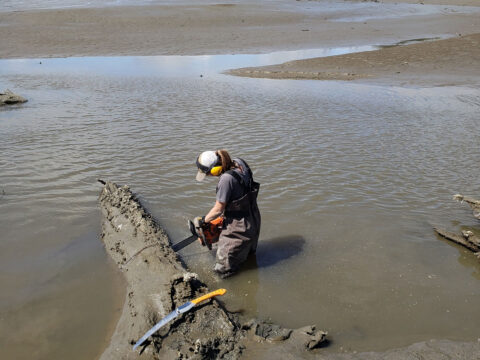
These local corrections could be improved by collecting more sampled material that fills spatial gaps and goes back further in time. At the workshop, researchers presented the exciting development that they were in the process of collecting annual radiocarbon measurements from Pacific geoduck clam shells off the Cascadian coastline to improve local marine reservoir knowledge. Geoducks can live more than 100 years and have annual growth rings that are sensitive to local climate and can therefore be cross dated to the exact year. Thus, a chronology of local climatic variation and marine radiocarbon abundance can be constructed using living and deceased specimens. Annual measurements of radiocarbon derived from marine bivalves, like the geoduck, offer new avenues to generate local marine reservoir corrections and improve age estimates for coseismic turbidity flows.
Putting It All Together
An imminent magnitude 9 megathrust earthquake on the CSZ poses one of the greatest natural hazards in North America and motivates diverse research across the Earth sciences. Continued development of multiple geochronologic approaches will help us to better constrain the timing of past CSZ earthquakes. And integrating earthquake age estimates with the understanding of rupture characteristics inferred from geologic evidence will help us to identify natural variability in past earthquakes and a range of possible future earthquake hazard scenarios.
Useful geochronologic approaches include using optically stimulated luminescence to date tsunami sand deposits (Figure 1) and determining landslide age estimates on the basis of remotely sensed land roughness [e.g., LaHusen et al., 2020]. Of particular value will be focuses on improving high-precision radiocarbon and dendrochronological dating of CSZ earthquakes, paired with precise estimates of subsidence magnitude, tsunami inundation from hydrologic modeling, inferred ground motion characteristics from sedimentological variations in turbidity deposits, and evidence of ground failure in subaerial, lake, and marine settings. Together, such lines of evidence will lead to better correlation of geologic records with specific earthquake rupture characteristics.
Ultimately, characterizing the recurrence of major earthquakes on the CSZ megathrust—which have the potential to drastically affect millions of lives across the region—hinges on the advancement and the integration of diverse geochronologic and geologic records.
Acknowledgments
We give many thanks to all participants in the USGS Cascadia Recurrence Workshop, specifically J. Gomberg, S. LaHusen, J. Padgett, B. Black, N. Nieminski, and J. Hill for their contributions.
References
Atwater, B. F., et al. (2014), Rethinking turbidite paleoseismology along the Cascadia subduction zone, Geology, 42(9), 827–830, https://doi.org/10.1130/G35902.1.
Atwater, B. F., et al. (2015), The Orphan Tsunami, 2nd ed., U.S. Geol. Surv., Reston, Va.
Garrison-Laney, C., and I. Miller (2017), Tsunamis in the Salish Sea: Recurrence, sources, hazards, in From the Puget Lowland to East of the Cascade Range: Geologic Excursions in the Pacific Northwest, GSA Field Guide, vol. 49, pp. 67–78, Geol. Soc. of Am., Boulder, Colo. https://doi.org/10.1130/2017.0049(04).
Goldfinger, C., et al. (2012), Turbidite event history — Methods and implications for Holocene paleoseismicity of the Cascadia Subduction Zone, U.S. Geol. Surv. Prof. Pap., 1661-F, https://doi.org/10.3133/pp1661F.
Heaton, T. J., et al. (2020), Marine20—The marine radiocarbon age calibration curve (0–55,000 cal BP), Radiocarbon, 62(4), 779–820, https://doi.org/10.1017/RDC.2020.68.
Howarth, J. D., et al. (2021), Calibrating the marine turbidite palaeoseismometer using the 2016 Kaikōura earthquake, Nat. Geosci., 14(3), 161–167, https://doi.org/10.1038/s41561-021-00692-6.
LaHusen, S. R., et al. (2020), Rainfall triggers more deep-seated landslides than Cascadia earthquakes in the Oregon Coast Range, USA, Sci. Adv., 6(38), eaba6790, https://doi.org/10.1126/sciadv.aba6790.
Melgar, D. (2021), Was the January 26th, 1700 Cascadia earthquake part of an event sequence?, EarthArXiv, https://doi.org/10.31223/X5XG78.
Melgar, D., et al. (2016), Kinematic rupture scenarios and synthetic displacement data: An example application to the Cascadia subduction zone, J. Geophys. Res. Solid Earth, 121, 6,658–6,674, https://doi.org/10.1002/2016JB013314.
Miyake, F., et al. (2012), A signature of cosmic-ray increase in AD 774–775 from tree rings in Japan, Nature, 486(7402), 240–242, https://doi.org/10.1038/nature11123.
Pearl, J. K., et al. (2020), A late Holocene subfossil Atlantic white cedar tree-ring chronology from the northeastern United States, Quat. Sci. Rev., 228, 106104, https://doi.org/10.1016/j.quascirev.2019.106104.
Wirth, E. A., and A. D. Frankel (2019), Impact of down-dip rupture limit and high-stress drop subevents on coseismic land-level change during Cascadia Megathrust earthquakes, Bull. Seismol. Soc. Am., 109(6), 2,187–2,197, https://doi.org/10.1785/0120190043.
Yamaguchi, D. K., et al. (1997), Tree-ring dating the 1700 Cascadia earthquake, Nature, 389(6654), 922–923, https://doi.org/10.1038/40048.
Author Information
Jessie K. Pearl, Earthquake Science Center, U.S. Geological Survey, Seattle, Wash.; and Lydia Staisch ([email protected]), Geology, Minerals, Energy, and Geophysics Science Center, U.S. Geological Survey, Portland, Ore.
Citation:
Pearl, J. K., L. Staisch (2021), Swipe left on the “big one”: Better dates for Cascadia quakes, Eos, 102, https://doi.org/10.1029/2021EO162183. Published on 20 August 2021.
Text not subject to copyright.
Except where otherwise noted, images are subject to copyright. Any reuse without express permission from the copyright owner is prohibited.