Deep within Earth’s interior, in the brittle lithosphere and in the viscous asthenosphere below it, magma collects and ultimately feeds erupting volcanoes on Earth’s surface. This magma holds keys to understanding the processes that create island chains like Hawaii.
However, scientists can map the mantle—or any part of Earth’s interior—only by using remote sensing techniques. The most important such technique is seismic tomography.
Were it not for seismology, we would be almost totally ignorant of the structure of the deep interior of Earth.
Similar to medical computerized tomography (CT) scanning, seismic tomography allows scientists to construct three-dimensional images of deep Earth from observations of seismic waves that have passed through it. The speed of these waves varies depending on the composition, phase, temperature, and pressure of the material through which they travel. Analyzing seismic waves can thus reveal details of these deep materials. Were it not for seismology, we would be almost totally ignorant of the structure of the deep interior of Earth.
The term “seismic tomography” was first coined by Adam Dziewonski and Don L. Anderson [Dziewonski and Anderson, 1984]. Over subsequent decades, the method has received substantial research funding, and the results have profoundly influenced our knowledge of Earth’s structure and dynamics.
Nevertheless, communicating the technique’s limitations between subdisciplines within Earth science remains a challenge. For reasons of space and other factors, published papers often tend to deemphasize important caveats [Foulger et al., 2013]. The bottom line is that scientists tend to underestimate pitfalls and often have disproportionate faith in results.
Teleseismic Tomography
Scientists apply seismic tomography on scales ranging from small crustal features a few kilometers across to the entire mantle, which is some 3000 kilometers thick. A method commonly used to study regions up to a few hundreds of kilometers wide and deep, referred to as teleseismic tomography, typically has a resolution on the order of 10 kilometers.
Teleseismic tomography analyzes the relative arrival times at surface seismometers of seismic waves from distant earthquakes that have traveled through the interior of Earth. Seismic waves slow down when traveling through some materials (e.g., iron-rich rocks, partially molten material, or hot rock), and as a result, some waves reach seismic monitoring stations slightly delayed. Scientists then convert these timing delays to information about wave speeds in the material through which the seismic waves passed.
Over the past 3 decades, scientists have made enormous progress in assembling software and data for teleseismic tomography. They have developed sophisticated inversion programs and established large equipment pools, enabling practitioners to deploy hundreds of stations in dense arrays. They have also densified national and global seismic networks and greatly improved their quality.
Thanks to this progress, model resolution significantly improved. Nevertheless, important challenges remain—some technical and some involving the way we communicate science.
Limitations of Teleseismic Tomography
Teleseismic tomography, although sensitive to horizontal variations in seismic wave speed, has virtually no ability to determine depth variations.
Teleseismic tomography, although sensitive to horizontal variations in seismic wave speed, has virtually no ability to determine vertical (depth) variations. This defect stems from relying exclusively upon rays that sample all depths in the same way. Arbitrary changes in wave speeds throughout a horizontal slab produce only minuscule variations in the predicted relative arrival times and thus are virtually undetectable. Among other things, this fact means that scientists cannot establish the vertical continuity of tomographic anomalies with certainty.
Another reason researchers cannot determine the true depth extent of imaged structures is that the incoming seismic waves arrive traveling steeply upward because they have been refracted toward the surface by the increase of wave speed with depth in Earth. The resulting strongly nonuniform ray distribution causes vertical smearing in tomographic images and gives distorted pictures that often contain spurious pipe-like features.
Compounding these problems, researchers commonly display structures derived as differences relative to one-dimensional models of the (unknown) local average wave speeds. This practice makes it difficult for readers to compare results from different regions [Bastow, 2012]. Alternatively, results may be shown relative to an assumed average global model, but this conceals major global features such as the seismic low-velocity zone, a layer in the shallow mantle thought to represent the asthenosphere [Thybo, 2006].
In all tomography methods, the strengths of calculated anomalies depend heavily on subjective choices of the inversion parameters used, such as those involving damping or smoothing. Despite this, researchers still commonly assume that the relative strengths of resulting anomalies are accurate.
Scientists often unjustifiably interpret these strengths as direct reflections of the temperature of material through which the seismic waves have passed. Widely used assumptions hold that high wave speeds correspond to cold, probably dense and sinking material and low velocities correspond to hot, rising material. However, these assumptions cannot be made because variations in composition and degree of contained partial melt (which is strongly related to hydrogen and carbon content) have larger effects on seismic wave speed than temperature. In some unusual cases where scientists checked the assumptions independently, these assumptions proved to be false [e.g., Trampert et al., 2004].
Researchers need an even greater leap of faith to deduce convective motion. Tomography can only reveal instantaneous (on a geological timescale) snapshots of the structure of the mantle, not its internal motion.
Difficulties in Assessing the Reliability of Results
Scientists make great efforts to assess the reliability of their results, but such assessments are not easy. Common tests of model integrity prove unreliable because they are limited to the mathematical approach used and fail to address several significant sources of error (Figure 1) [Foulger et al., 2014; Léveque et al., 1993; van der Hilst et al., 1993].
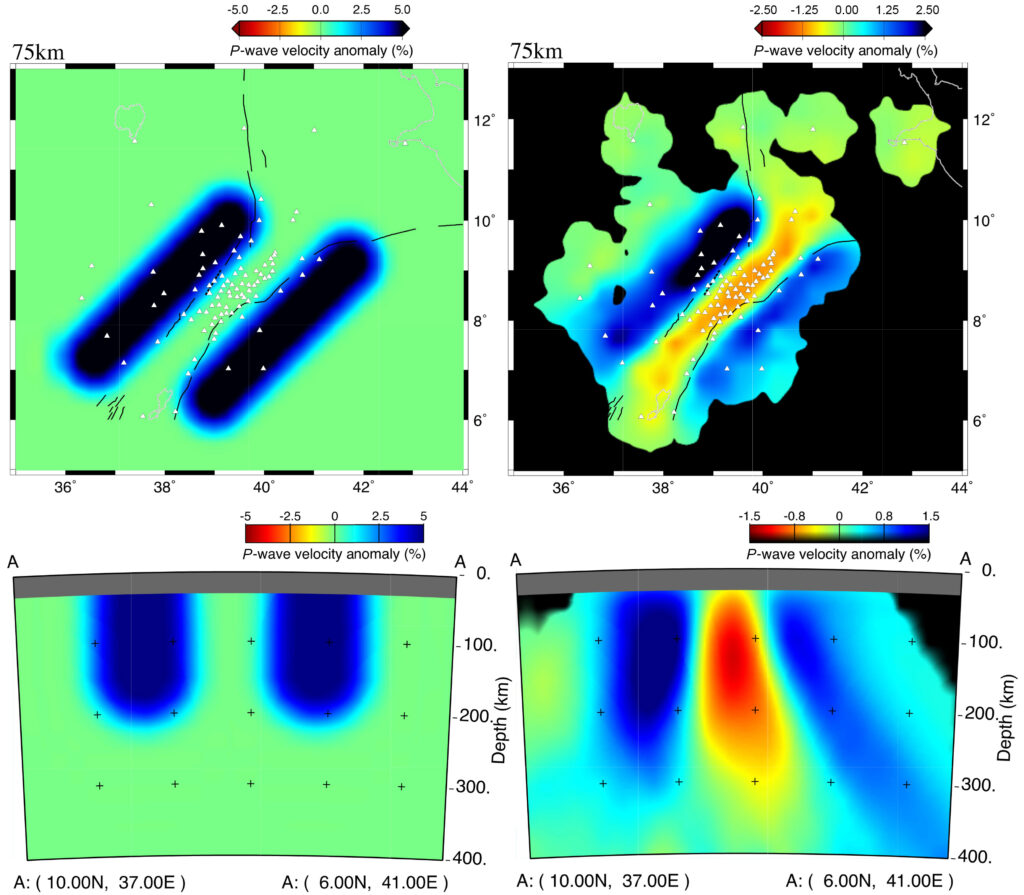
The scientific community cannot assess the absolute accuracy of a result because no method exists that can provide superior results against which the results of teleseismic tomography can be compared. Furthermore, scientists cannot really know whether the method as used answers the question that the researchers intended (known as “epistemic” uncertainty).
The next best thing involves examining the repeatability of independent studies. However, scientists typically don’t perform multiple tomography studies covering the same locality. Even if they do, experiments are not usually completely independent. Such experiments often draw data from the same pool, and the mathematical methods and computer programs used may be related.
The Mantle Plume Controversy: Do Plumes Even Exist?
A few volcanic regions, however, have been the subjects of multiple tomography studies. Scientists find these areas particularly interesting—some postulate that they are fueled by an elusive theoretical phenomenon: deep mantle plumes.
These regions, which include Hawaii and Yellowstone, are puzzling because they lie far from the boundaries of tectonic plates where most igneous activity occurs. The plume hypothesis proposes that they are fed by columns of hot, solid rock a few tens or hundreds of kilometers in diameter rising from the core-mantle boundary and independent of the convection involved in plate tectonics. Scientists expect that deep mantle plumes, if they exist, should be visible in tomographic images as columnar regions of low seismic wave speed.
During the past decade, new observations have thrown the existence of mantle plumes into serious doubt. Predictions of the hypothesis—such as the updoming of Earth’s surface and flood volcanism when a plume eruption begins—seldom are confirmed by observation [Foulger, 2010]. As a result, many ongoing studies attempt to scrutinize the hypothesis against observations.
Hawaii, the archetypal candidate for the location of a deep mantle plume, thus becomes an important target for observations. If the existence of a plume under Hawaii could be confirmed or ruled out, it could help settle the debate regarding whether or not plumes exist in general.
Unfortunately, Hawaii is extraordinarily poorly placed for study, with a relatively small landmass and few nearby earthquake sources. Teleseismic tomography experiments typically cannot probe deeper than the aperture of the seismometer network used. As a result, only the upper few hundred kilometers of the mantle beneath Hawaii can be reliably imaged; seismic tomography cannot reach down a few thousand kilometers, where plumes are thought to originate. Recent studies exhibit little repeatability, even at shallow depths below Hawaii (Figure 2).
![Fig. 2. Lack of repeatability in recent studies of Hawaii: (left) from Li et al. [2008], showing a low-velocity body, interpreted as a plume, tilting from the Big Island of Hawaii to the northwest beneath the Hawaiian island chain; (right) from Wolfe et al. [2009], showing a similar low-velocity body, also interpreted as a plume, except tilting in the exact opposite direction—from beneath the Big Island of Hawaii to the southeast.](https://eos.org/wp-content/uploads/2015/08/15-0144_FOULGER_4861-F02_EDITED_RGB_FINAL_WEB-1024x838.jpg)
The Yellowstone Opportunity
Yellowstone is in a very different situation. It lies in a continental setting, and researchers can deploy extensive, optimized seismic networks relatively easily. As a result, scientists have targeted it for numerous ambitious experiments since the 1970s.
The culmination of these projects is the roughly $500 million, 2000-site USArray seismic network, which covers the entire United States. Many tomographic studies of the Yellowstone region use the superb data it has collected (see Pavlis et al. [2012] for a review). These studies provide the best opportunity currently available to study the repeatability of teleseismic tomography for a proposed plume region.
The results show broad agreement regarding bodies on a scale of several tens of kilometers. These include the sinking Farallon slab—a “lost” tectonic plate that became completely subsumed within the mantle over the past 15 million years or so. Today the slab exhibits high wave speeds, partly because it is colder and more rigid than the surrounding mantle.
If teleseismic tomography cannot settle the debate at an ideally located and monitored place such as Yellowstone, the question arises as to whether it can do so anywhere.
Researchers also see a strong, shallow, low–wave speed anomaly beneath the Eastern Snake River Plain and Yellowstone and high wave speeds farther east beneath the central United States. However, repeatability deteriorates for smaller bodies and with increasing depth from about 200 to 1000 kilometers.
Remarkably, because of the lack of repeatability between results at depths greater than 200 kilometers, this work has brought no consensus regarding the shape, location, continuity, or depth extent of seismic wave speed anomalies directly beneath Yellowstone, nor has it resolved whether a mantle plume exists below the crust [Foulger et al., 2015]. If teleseismic tomography cannot settle the debate at an ideally located and monitored place such as Yellowstone, the question arises as to whether it can do so anywhere.
The Future of Teleseismic Tomography
Seismic tomography—an immensely powerful tool that deserves its recent popularity—shines a light on the mantle, influencing almost every branch of Earth science.
However, the greatest remaining challenge may lie not in doing better tomography but in appreciating its limitations and living with the results. Tomography models need to be shared within the Earth science community in a way that communicates not only the results’ robust features but also their real errors [Panza et al., 2007].
Seismic tomography results also need to be interpreted jointly with other data, including gravity studies and tectonic reconstructions. The research community needs such interdisciplinary work to reduce ambiguities in interpretation.
Researchers often turn to the geochemistry of surface samples to back up interpretations, but this approach is much less helpful than commonly assumed. The geochemical compositions of igneous rocks at the surface cannot be used to infer deep mantle plumes or to assess the composition and convective motion of the deep mantle. Moreover, any attempts at geochemical “ground truthing” have only limited power to estimate the temperature of the melting mantle sources that feed surface volcanism [Lustrino and Anderson, 2015].
Understanding what is not reliable in a seismic tomography image and interpreting what is reliable in terms of geology thus remain perhaps our most exciting current challenges.
References
Bastow, I. D. (2012), Relative arrival-time upper-mantle tomography and the elusive background mean, Geophys. J. Int., 190, 1271–1278.
Dziewonski, A. M., and D. L. Anderson (1984), Seismic tomography of the Earth’s interior, Am. Sci., 72, 483–494.
Foulger, G. R. (2010), Plates vs Plumes: A Geological Controversy, 328 pp., Wiley-Blackwell, Chichester, U. K.
Foulger, G. R., et al. (2013), Caveats on tomographic images, Terra Nova, 25, 259–281.
Foulger, G. R., et al. (2014), Caveats on tomographic images, paper presented at European Geosciences Union General Assembly, Vienna, Austria, 27 April to 2 May.
Foulger, G. R., R. L. Christiansen, and D. L. Anderson (2015), The Yellowstone ‘hot spot’ track results from migrating Basin Range extension, in The Interdisciplinary Earth: A Volume in Honor of Don L. Anderson, edited by G. R. Foulger, M. Lustrino, and S. D. King, Geol. Soc. of Am., Boulder, Colo., in press.
Léveque, J. J., L. Rivera, and G. Wittlinger (1993), On the use of the checker-board test to assess the resolution of tomographic inversions, Geophys. J. Int., 115, 313–318.
Li, C., R. D. van der Hilst, E. R. Engdahl, and S. Burdick (2008), A new global model for P wave speed variations in Earth’s mantle, Geochem. Geophys. Geosyst., 9, Q05018, doi:10.1029/2007GC001806.
Lustrino, M., and D. L. Anderson (2015), The mantle isotopic printer: Basic mantle plume geochemistry for seismologists and geodynamicists, in The Interdisciplinary Earth: A Volume in Honor of Don L. Anderson, edited by G. R. Foulger, M. Lustrino, and S. D. King, Geol. Soc. of Am., Boulder, Colo., in press.
Panza, G. F., A. Peccerillo, A. Aoudia, and B. Farina (2007), Geophysical and petrological modelling of the structure and composition of the crust and upper mantle in complex geodynamic settings: The Tyrrhenian Sea and surroundings, Earth Sci. Rev., 80, 1–46.
Pavlis, G. L., K. Sigloch, S. Burdick, M. J. Fouch, and F. L. Vernon (2012), Unraveling the geometry of the Farallon plate: Synthesis of three-dimensional imaging results from USArray, Tectonophysics, 532–535, 82–102.
Thybo, H. (2006), The heterogeneous upper mantle low velocity zone, Tectonophysics, 416, 53–79.
Trampert, J., F. Deschamps, J. Resovsky, and D. Yuen (2004), Probabilistic tomography maps chemical heterogeneities throughout the lower mantle, Science, 306, 853–856.
van der Hilst, R., E. R. Engdahl, and W. Spakman (1993), Tomographic inversion of P and pP data for aspherical mantle structure below the northwest Pacific region, Geophys. J. Int., 115, 264–302.
Wolfe, C. J., S. C. Solomon, G. Laske, J. A. Collins, R. S. Detrick, J. A. Orcutt, D. Bercovici, and E. H. Hauri (2009), Mantle shear-wave velocity structure beneath the Hawaiian hot spot, Science, 326, 1388–1390.
Author Information
Gillian R. Foulger, Department of Earth Sciences, Durham University, Durham, U. K.; email: [email protected]; Giuliano F. Panza, Department of Mathematics and Geosciences, University of Trieste, Trieste, Italy; also at Structure and Non-Linear Dynamics of the Earth, International Center for Theoretical Physics, Trieste, Italy, and the Institute of Geophysics, China Earthquake Administration, Beijing, China; Irina M. Artemieva, Department of Geography and Geology, University of Copenhagen, Copenhagen, Denmark; Ian D. Bastow, Department of Earth Science and Engineering, Imperial College, London, U. K.; Fabio Cammarano, Department of Geography and Geology, University of Copenhagen, Copenhagen, Denmark; Carlo Doglioni, Dipartimento Scienze della Terra, Università Sapienza, Rome, Italy; John R. Evans, Earthquake Science Center, U.S. Geological Survey, Santa Cruz, Calif; Warren B. Hamilton, Department of Geophysics, Colorado School of Mines, Golden; Bruce R. Julian, Department of Earth Sciences, Durham University, Durham, U. K.; Michele Lustrino, Dipartimento di Scienze della Terra, Università degli Studi di Roma La Sapienza, Rome, Italy; Hans Thybo, Department of Geography and Geology, University of Copenhagen, Copenhagen, Denmark; and Tatiana B. Yanovskaya, Department of Physics of the Earth, Saint Petersburg State University, Saint Petersburg, Russia
Citation: Foulger, G. R., G. F. Panza, I. M. Artemieva, I. D. Bastow, F. Cammarano, C. Doglioni, J. R. Evans, W. B. Hamilton, B. R. Julian, M. Lustrino, H. Thybo, and T. B. Yanovskaya (2015), What lies deep in the mantle below?, Eos, 96, doi:10.1029/2015EO034319. Published on 25 August 2015.
Text © 2015. The authors. CC BY-NC 3.0
Except where otherwise noted, images are subject to copyright. Any reuse without express permission from the copyright owner is prohibited.