Several decades ago, when the concentration of carbon dioxide (CO2) in the atmosphere was well below 400 parts per million, climate scientists began warning of the negative consequences for Earth’s climate of burning fossil fuels. From those early warnings, a consensus emerged that carbon emissions would need to be lowered (and eventually zeroed out) to avoid dangerous consequences of global warming such as extreme heat, stronger storms, and more intense floods and droughts.
Today the atmospheric CO2 concentration is well over 400 parts per million and still rising, and a plethora of research and recent severe weather events point to the fact that these dangerous consequences are already happening. Governments have set ambitious goals to curb emissions, and some progress is being made, but serious questions and concerns about the slow pace of this progress abound.
For each approach to carbon dioxide removal, questions remain over the scale of impact it can have in drawing down and safely storing atmospheric carbon.
Effectively lowering atmospheric carbon levels will require a range of actions, from individuals making hard decisions about lifestyle changes to international cooperation to pursue solutions from a diverse menu of options. Among the options under consideration are methods for deliberate carbon dioxide removal (CDR) from the atmosphere, once considered a last resort. Many approaches to CDR, both on land and at sea, are in various stages of testing and development. Each has upsides and downsides, for example, with respect to costs and potentially negative secondary effects. And for each, questions remain over the scale of impact it can have on drawing down and safely storing atmospheric carbon.
Here we outline an alternative CDR approach, one inspired by a natural carbon-sequestering process at the seafloor, that in theory could remove substantial amounts of atmospheric carbon. Significant questions and concerns would need to be answered about this approach, too, but considering its vast potential, we think it is worth investigating.
The Current Menu of Carbon Dioxide Removal Options
The field of CDR is exploding as demand for cost-effective strategies exceeds supply. The most familiar approaches look to store organic carbon in biomass, on land, and in the oceans. Efforts to restore natural habitats with capacity for carbon storage are laudable. However, this organic carbon is vulnerable to oxidation and rerelease into the atmosphere, most notably through combustion in wildfires and decay of drought-stricken vegetation. At sea, such efforts are pursued through seaweed cultivation and nutrient fertilization, for example, although it is unclear how much of the new biomass created is ultimately stored away or for how long.
Carbon dioxide may also be captured by using acid-base chemistry, and then sequestered in rock (e.g., depleted oil reservoir formations) or in the ocean. However, such approaches also run into challenges of achieving permanent storage, raising questions about the security and potential for rerelease of sequestered carbon to the atmosphere.
Ocean alkalinity enhancement holds considerable promise because the ocean’s capacity for storing bicarbonate is ample on the relevant time frame.
Converting CO2 to mineral carbonate affords a mechanism for permanent storage [Kelemen and Matter, 2008]. Natural reactions known as the Ebelmen-Urey reactions [Pierrehumbert, 2010], in which silicate minerals such as forsterite (Mg2SiO4; a form of olivine) react with CO2 to yield carbonate minerals and silica (e.g., Mg2SiO4 + 2CO2 → 2MgCO3 + SiO2), are considered to have acted as a planetary thermostat over most of Earth’s history because of their dependence on temperature and related feedbacks. These reactions have, in the past, gradually pulled Earth out of warm greenhouse climates by lowering atmospheric CO2, and they will eventually erase the anthropogenic carbon spike, although not on a timescale relevant to human civilization.
In the meantime, humanity may need to implement CDR on a vast scale to compensate for the extraction and combustion of fossil fuels over the past century. Ocean alkalinity enhancement (OAE), in which the addition of ions like Mg2+ and Ca2+ (sourced from materials such as olivine or lime) to the ocean drives more dissolution of atmospheric CO2 to form bicarbonate (HCO3–), holds considerable promise, because the ocean’s capacity for storing bicarbonate is ample on the relevant time frame [Renforth and Henderson, 2017]. Indeed, OAE approaches—often involving materials dispersed at the ocean surface—are being studied, though they, too, face questions about their large-scale feasibility. Are there other marine settings where OAE could be pursued on a broad scale and with durable results?
Transform Faults Offer a Transformative Approach
Earth’s mantle, constituting more than 80% of the planet’s volume, is a vast reservoir of ultramafic (low-silica) rock. In concept, a small fraction of this rock—minimally about 600 cubic kilometers if completely converted to carbonate—could neutralize the entire slug of Industrial Age fossil carbon in the atmosphere.
Oceanic transform faults and their fracture zone extensions present tectonic settings where such reactive mantle rocks, which are typically buried under kilometers of crust, are exposed at Earth’s surface. The discovery of transform faults—which connect the divergent plate boundaries located at mid-ocean spreading centers—was key to unleashing the plate tectonic revolution in the 1960s [Karson, 2020]. And today, the co-occurrence of the right rocks and high-relief bathymetry presents an optimal combination of chemical and gravitational disequilibria, suggesting a potential for large-scale CDR found nowhere else on Earth (Figure 1).
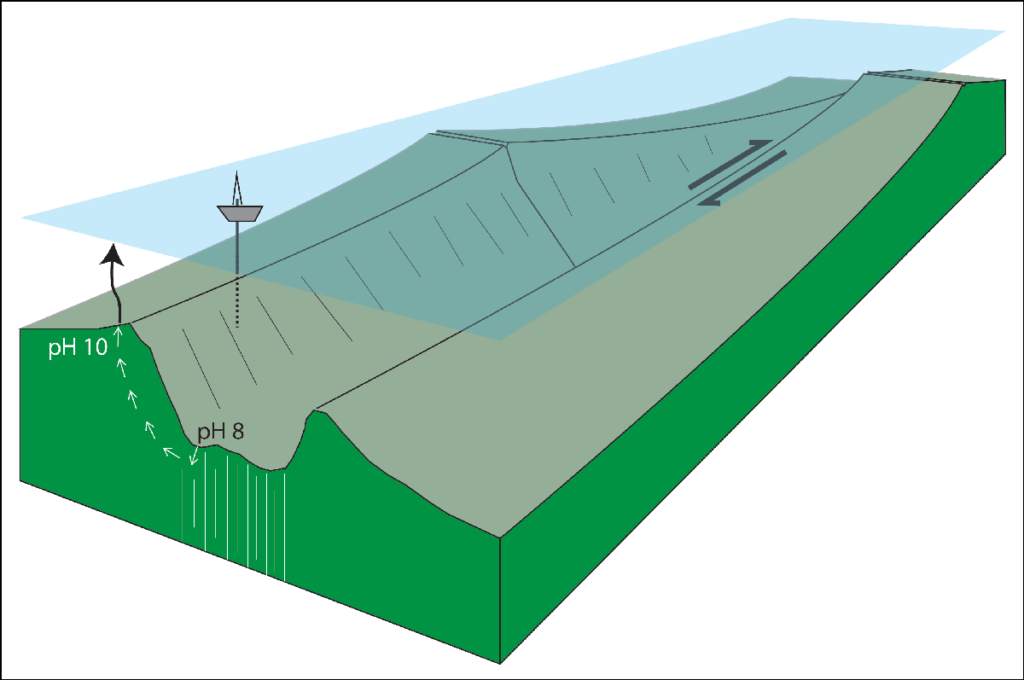
Especially at magma-poor slow spreading (<4 centimeters per year) plate boundaries, transform fault settings feature relatively fast reacting magnesium silicate minerals in abundance [Kelemen et al., 2020]. The scale of transform fault valleys dwarfs that of terrestrial erosional features such as the Grand Canyon. Submarine valley walls are prone to mass wasting, which exposes fresh surfaces of reactive silicate minerals. Locally, the motion of almost-horizontal detachment faults results in portions of crust sliding off the underlying mantle, allowing further exposure of ultramafic rock on the seafloor.
Active fracturing, needed for water-rock reactions to yield alkaline solutions, is widespread. Slow velocities of seismic waves observed along oceanic transform faults imply that water penetrates to depths of more than 30 kilometers [Wang et al., 2022]. Because of differential cooling in the seafloor rock in these settings, the fracture zone extensions of transform plate boundaries also continue to experience differential vertical movement and fresh fracturing. In addition, active faults exposing reactive mantle rock are locally present along ridge crests and along trench walls.
Suburbanize Lost City?
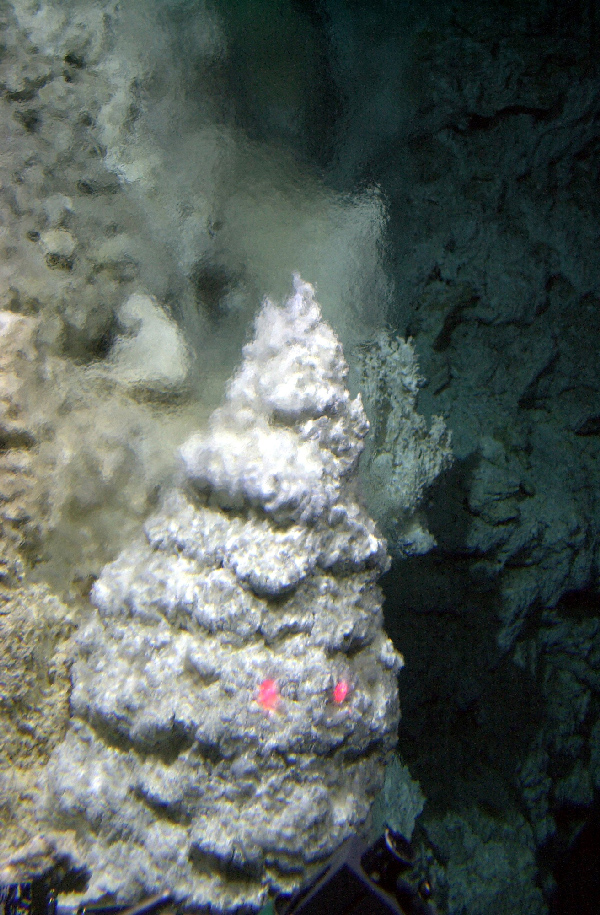
Oceanic transform fault settings are known to host low-temperature hydrothermal systems that sequester dissolved CO2 by precipitating mineral carbonate [Kelley et al., 2007]. A prime example, the Lost City hydrothermal field (LCHF), sits at about 30°N latitude and 15 kilometers west of the Mid-Atlantic Ridge on the Atlantis Massif. Here, seafloor vents release high-pH, alkaline fluids that react with seawater to precipitate towers over 60 meters tall made of carbonate (e.g., CaCO3) and brucite (Mg[OH]2).
Low-temperature hydrothermal circulation at the LCHF is linked to the exothermic hydration of olivine and related minerals by water percolating beneath the seafloor (e.g., 2Mg2SiO4 + H2O + 2H+ → Mg3Si2O5(OH)4 + Mg2+). This reaction forms serpentine-group minerals (e.g., Mg3Si2O5(OH)4), the primary minerals in serpentinite rock. The hydrothermal fluids then rise through a permeable system of fractured rock. Mixing of the warm, alkaline vent fluids (pH > 10) with seawater shifts the carbonate equilibria locally to favor carbonate precipitation.
Imagine if it were feasible to enhance this natural CDR process by deliberately expanding low-temperature hydrothermal systems such as Lost City and delivering the alkaline fluids to the ocean surface to simultaneously reverse anthropogenic ocean acidification and draw down atmospheric CO2. It may seem an audacious and technically demanding feat, but the core technologies needed are already available.
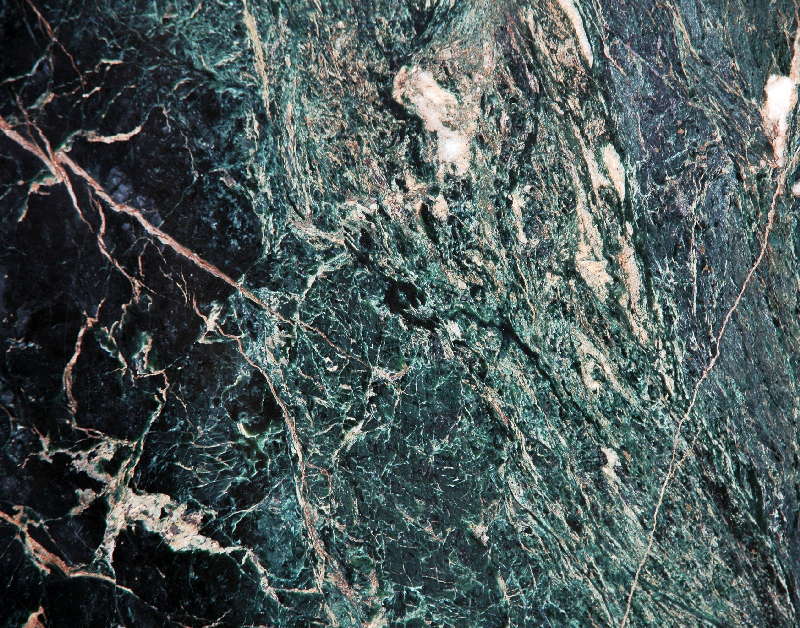
Drilling and hydrofracturing at sites of active mass wasting would create fresh reactive mineral surfaces and promote serpentinization, further cracking, and the production of greater volumes of alkaline, high-pH hydrothermal fluids. Then the fluids could be either pumped or directed to rise buoyantly through insulated pipelines to raise the alkalinity of the surface ocean mixed layer. By comparison with the existing fossil fuel infrastructure that crisscrosses the continents and seafloor, piping these fluids to the surface should be eminently doable. As for surface infrastructure, mothballed fleets of aircraft carriers—presumably powered by nonfossil sources of energy such as nuclear or wind—might serve as drilling platforms. Earth’s transform fault scarps cover on the order of 100,000 square kilometers, an area likely more than sufficient for this approach to CDR [e.g., Kelemen et al., 2020].
Even if the fundamental technology and scale of exposed mantle material are available, however, there are practical scientific questions to address. For example, additional research would be needed to understand the relative importance of negative and positive feedbacks in low-temperature hydrothermal settings [Kelemen et al., 2020]. Negative feedbacks might include “clogging,” where the precipitation of secondary minerals inhibits permeability and the production of alkaline fluids. Positive feedbacks that keep these systems going, meanwhile, are evidenced by both the long lives of vents and the pervasiveness of fractured and altered rock.
There are also potential kinetic issues to contend with—although the chemistry favors increased consumption of CO2 in the ocean, the pace of the reactions may be too slow to matter on human timelines. Several options have been explored to accelerate rates of CO2-consuming reactions. Kelemen and Matter [2008], for example, showed that the rate of olivine carbonation increases a millionfold above typical rates at the optimal reaction temperature of 185°C (365°F) and high partial pressures of CO2. Electrochemical strategies to accelerate the process have also been explored [e.g., Rau et al., 2013].
The Trouble with Methane
The notion of intentionally expanding hydrothermal systems and piping fluids to the ocean surface raises distinct concerns. High on the list is methane.
Beyond questions of its technical feasibility, the notion of intentionally expanding hydrothermal systems and piping fluids to the ocean surface raises distinct concerns. High on the list is that methane, a potent greenhouse gas, is a ubiquitous product of serpentinization. What role do carbon-free minerals play in the formation of carbon-rich methane?
In serpentinization reactions, mantle olivine, a solid solution of typically 90% forsterite (Mg2SiO4) and 10% fayalite (Fe2SiO4), releases reduced iron (Fe2+), which is the culprit. Water oxidizes the reduced iron, forming molecular hydrogen (H2) in the process (i.e., 3Fe2SiO4 + 2H2O → 2Fe3O4 + 3SiO2 + 2H2). This hydrogen then converts any oxidized carbon (e.g., CO2) present to methane (i.e., 4H2 + CO2 → CH4 + 2H2O). It clearly would be undesirable to create or enlarge methane sources and have the gas end up in the atmosphere.
The news might not be all bad, however. Both hydrogen and methane gases are energy sources—the former a clean energy source. Harvesting the gases could help to meet continuing demand for conventional energy and growing demand for clean energy while also helping to finance drilling and CDR infrastructure. In an alternate, economically focused framing, the main goal for expanding hydrothermal vent systems as described could even be to produce and market income-generating sources of hydrogen gas, with CDR as a beneficial by-product.
Harvesting these gases in the deep ocean could of course prove challenging. At Lost City, the carbonate capping rock at the seafloor helps to focus the flows of venting fluids. Whether an artificial infrastructure would similarly focus the gases produced without excessive leakage is an open question.
What Else Could Go Wrong?
Other concerns, apart from the potential escape of excess methane, exist as well. Low-temperature hydrothermal systems such as Lost City stand in sharp contrast to high-temperature hydrothermal systems, which are common along mid-ocean ridges. These high-temperature systems, in which dissolved magnesium is in effect traded for protons (i.e., Mg2+ → 2H+), vent acidic solutions that subtract from the ocean’s alkalinity budget, so inadvertently increasing fluid fluxes from these systems would be counterproductive. Clearly, proximity to sites of high heat flow is to be avoided.
Drilling above fault scarps could also conceivably trigger mass wasting events and tsunamis, a potential hazard that has been studied in tectonically active regions such as the Puerto Rico Trench.
Activities associated with the proposed CDR approach could also disrupt seafloor and ocean surface habitats. Such disruption is a major concern with seafloor mining efforts, and substantial efforts would be required to avoid damaging seafloor ecosystems for the sake of CDR. To minimize disruptions in surface ocean habitats, a delivery system could be engineered to remove problematic solutes and to dilute the alkaline vent fluids before they were mixed with surface waters.
Dispelling a Wicked Problem
The Pandora’s box of fossil energy has created the “wicked” problem of climate change, which has no simple or single solution [Incropera, 2015]. The transition away from fossil energy sources is underway, but even if carbon emissions disappeared tomorrow, an excess of CO2 would long remain in our skies.
The natural CDR process that occurs at oceanic transform fault settings, if it could be harnessed, represents a potentially transformative solution.
No perfect options for removing excess carbon exist—all methods face questions over their safety, durability, and efficacy at large scale. The natural CDR process that occurs at oceanic transform fault settings, if it could be harnessed to draw down atmospheric CO2 and raise surface ocean pH, represents a potentially transformative solution. These settings combine optimal chemical and gravitational disequilibria at the scale needed—the right rocks ready to fracture are present in effectively unlimited quantity. If reduced gases like hydrogen and methane could be harvested for energy without fear of leakage, the approach could be financially sustainable.
Geoengineering solutions can have unintended consequences, so a cautious approach is in order. We suggest that more research is needed to investigate the technical feasibility of the CDR approach outlined here and identify possible test sites along transform faults for pilot drilling experiments. A system of governance to monitor and regulate research, testing, and development of marine CDR is needed as well.
So is this a potentially planet-saving idea worth exploring, or an intriguing but distracting one? We welcome input from the Earth sciences community and beyond. Regardless of one’s take, it’s becoming more and more clear that ambitious ideas are needed to speed progress toward mitigating the effects of global warming and dispel the wicked problem we’re facing.
Acknowledgments
We thank Jeffrey Karson and Dwight Bradley for helpful input. This article is the outcome of a reading group for geology majors offered at the University of Maine at Farmington during spring semester 2023.
References
Incropera, F. P. (2015), Climate Change: A Wicked Problem—Complexity and Uncertainty at the Intersection of Science, Economics, Politics, and Human Behavior, 337 pp., Cambridge Univ. Press, Cambridge, U.K., https://doi.org/10.1017/CBO9781316266274.
Karson, J. A. (2020), Oceanic transform faults, in Encyclopedia of Geology, 2nd ed., vol. 3, edited by D. Alderton and S. A. Elias, pp. 930–946, Elsevier, Amsterdam, https://doi.org/10.1016/B978-0-12-409548-9.12490-X.
Kelemen, P. B., and J. Matter (2008), In situ carbonation of peridotite for CO2 storage, Proc. Natl. Acad. Science U. S. A., 105(45), 17,295–17,300, https://doi.org/10.1073/pnas.0805794105.
Kelemen, P. B., et al. (2020), Engineered carbon mineralization in ultramafic rocks for CO2 removal from air: Review and new insights, Chem. Geol., 550, 119628, https://doi.org/10.1016/j.chemgeo.2020.119628.
Kelley, D. S., et al. (2007), The Lost City hydrothermal field revisited, Oceanography, 20(4), 90–99, https://doi.org/10.5670/oceanog.2007.09.
Pierrehumbert, R. T. (2010), Principles of Planetary Climate, 674 pp., Cambridge Univ. Press, Cambridge, U.K., https://doi.org/10.1017/CBO9780511780783.
Rau, G. H., et al. (2013), Direct electrolytic dissolution of silicate minerals for air CO2 mitigation and carbon-negative H2 production, Proc. Natl. Acad. Sci. U. S. A., 110(25), 10,095–10,100, https://doi.org/10.1073/pnas.1222358110.
Renforth, P., and G. Henderson (2017), Assessing ocean alkalinity for carbon sequestration, Rev. Geophys., 55(3), 636–674, https://doi.org/10.1002/2016RG000533.
Wang, Z., et al. (2022), Deep hydration and lithospheric thinning at oceanic transform plate boundaries, Nat. Geosci., 15, 741–746, https://doi.org/10.1038/s41561-022-01003-3.
Author Information
Doug Reusch ([email protected]), Kayleigh Brisard, Gil Hamilton, and Carson Theriault, University of Maine at Farmington