Los Angeles, Calif., is one of the 10 largest cities in the world that have been historically shaken by damaging earthquakes [Bilham, 2009]. The 1994 magnitude 6.7 Northridge earthquake, for example, sparked fires and collapsed roadways and buildings across the region. And although it caused no significant damage in Los Angeles, shaking from the Ridgecrest earthquake sequence that struck to the city’s north this past July served as a recent reminder of the city’s seismic vulnerability. Little doubt remains whether a future large earthquake will strike this region: The question is only when. Los Angeles therefore holds a special place in our existing understanding of—as well as in efforts to further illuminate—how best to mitigate natural hazards and their impacts on large populations.
The greater Los Angeles area—a megacity by the United Nations’ definition—is the second largest urban area in the United States, one of its fastest growing regions, and the third largest city in the world based on combined statistical area. Here the seismic hazard is driven by the potential proximity of large earthquakes and complicated local structure. Sources of potentially damaging earthquakes in the LA area include the southern San Andreas Fault, located roughly 60 kilometers northeast of the city, as well as the series of faults that lies below the area and just offshore. Meanwhile, the collection of complex sedimentary basins underlying the area is known to amplify the motions from seismic waves [e.g., Graves et al., 2011; Lovely et al., 2006].
Through the ShakeOut scenario, CyberShake, and other similar efforts, scientists are working to improve estimates of the ground shaking that would result from a large earthquake in this region. A plausible event detailed in the original ShakeOut scenario [Jones et al., 2008] is a magnitude 7–8 earthquake on the southern San Andreas Fault that causes large ground motions in downtown Los Angeles. One estimate of the ground motions in such a scenario, based on studying ambient noise correlations (correlations in background seismic signals) between seismic stations located on the San Andreas Fault and in downtown Los Angeles, suggests that these motions could be approximately 4 times larger than those predicted by current numerical simulations [Denolle et al., 2014]. This indicates that our assessments of risk could underestimate the potential damage due to this type of earthquake.
The low seismic velocities and concave shapes of these basins—the San Gabriel and the San Bernardino—tend to trap energy and channel it toward the downtown Los Angeles area, which leads to larger ground motions.
The discrepancy between the different methods appears to stem from the fact that the northern basins in the Los Angeles area are not well characterized by the current 3-D seismic velocity models used in the computer simulations. Instead of allowing seismic energy to disperse into the surrounding region, the low seismic velocities and concave shapes of these basins—the San Gabriel and the San Bernardino—tend to trap energy and channel it toward the downtown Los Angeles area, which leads to larger ground motions [e.g., Olsen et al., 2006].
Borehole and seismic reflection data in these basins are sparse, however, in part because oil companies—which have historically collected much of this sort of data—have not explored these basins as extensively as they have the Los Angeles Basin itself. This data shortage makes it difficult to determine precisely the shapes and seismic velocities of the basins, which hampers accurate earthquake hazard assessments. Additional data and improvements in the 3-D seismic velocity model used to simulate ground motions are thus of fundamental importance.
Volunteers Deploy Seismic Sensors
Starting in 2017, we set out to better determine the shapes and seismic velocities of the northern basins. We deployed dense 2-D seismic arrays across the San Gabriel and San Bernardino Basins (Figure 1), along with 20 additional seismic broadband instruments.
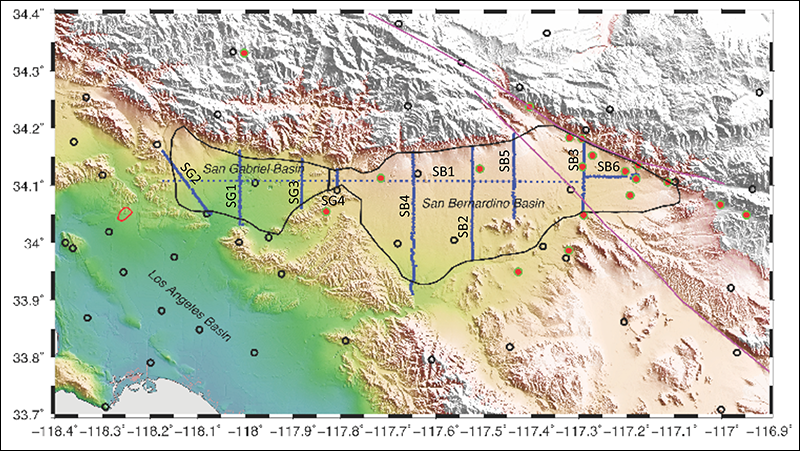
These surveys are possible only because of a new type of seismic instrumentation that was first used by oil companies in Los Angeles in 2011.
The data from these Basin Amplification Seismic Investigation (BASIN) surveys will be used to construct a 3-D model that should better predict the strong ground motions in downtown Los Angeles from events on the San Andreas Fault. These surveys are possible only because of a new type of seismic instrumentation—a compact, autonomous unit containing a standard 5-hertz three-component geophone, a battery, and a GPS clock—that was first used by oil companies in Los Angeles in 2011 [Lin et al., 2013].
The BASIN surveys described here represent a new type of deployment that might be called “urban seismology.” The strategy we pursued involved installing instruments in linear arrays, with two-person teams deploying 16–20 stations each along portions of instrument lines. The teams were given maps marked with assigned points and were instructed to place a single instrument within a half-block radius of each point. They looked to site instruments in viable private residences or businesses or, if none were available, in median strips along roads or open fields. Whenever possible, sensors were completely buried in a 20-centimeter hole to minimize noise and to keep the instruments hidden.
The deployments involve considerable interaction with the public in seeking permission to place the sensors on private property. We generally have a high success rate if residents are at home and answer the door, and for this reason we usually deploy on weekends. Our deployment teams have included approximately 60 volunteers who span a diverse range of ethnicities, genders, and careers and range in age from high school students to retirees.
There is a certain level of risk that these instruments will be lost or damaged—to date we have lost seven sensors, presumably because of theft. This is about 1% of the instruments we’ve deployed, which we consider an acceptable rate and inevitable with this type of survey. We also suspect that a couple of instruments were disturbed by coyotes.
Initially, we thought that the noisy environment of the basins would preclude effective recording of distant events, but that is not the case.
We have completed 9 sensor lines of a planned total of 10 lines. These 9 lines comprise 482 sensor sites, which have generated some 4 terabytes of data at 250 samples per second. The average station spacing in the in-line direction is approximately 250 meters, and the stations remained operational for ~35 days on the basis of battery life.
Structure from Data
We plan to use receiver functions—a technique to enhance seismic waves reflected off interfaces between layers in the subsurface—determined from moderate and large teleseismic earthquakes as far away as Fiji to determine the crustal structure beneath the sensor lines. Initially, we thought that the noisy environment of the basins would preclude effective recording of distant events, but that is not the case (Figure 2). The sensors along lines SG1, SG2, and SB4 clearly show the structure, including the Moho (the boundary between Earth’s crust and mantle) and interfaces above this boundary [Liu et al., 2018]. The sediment-basement interface (the bottom of the basins) is also well defined.
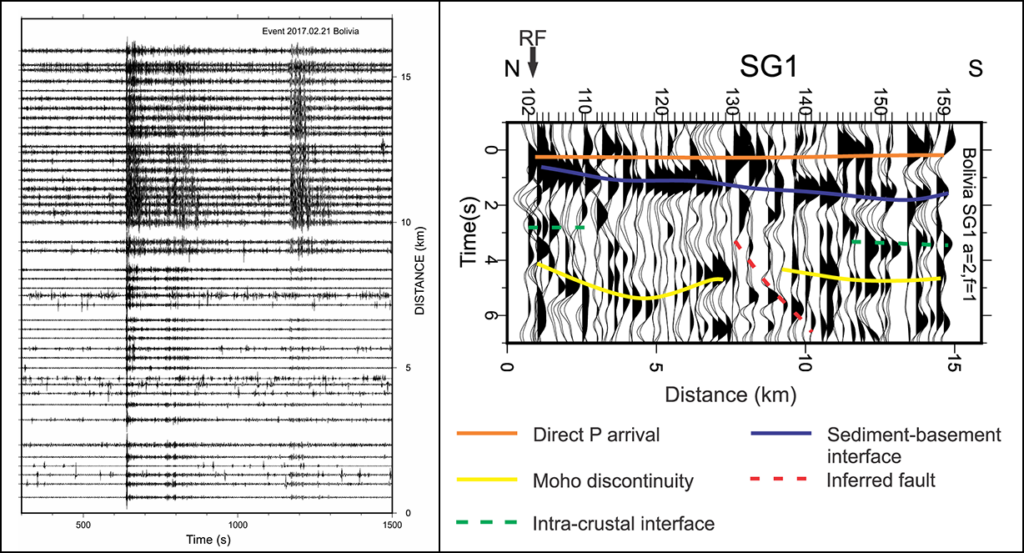
Determining a basin’s effectiveness in channeling seismic energy is contingent upon determining the basin’s shape and its shear wave velocity. To measure shear wave velocity, we plan to use the analysis of surface waves determined from ambient noise correlations, which has been shown to be effective in the Los Angeles region [Lin et al., 2013].
Figure 3 shows an example where Rayleigh and Love surface waves can be seen in the correlations. These types of waves have the largest amplitudes and thus produce the strongest ground motion. Also, Rayleigh and Love waves are most easily seen in correlations and thus are very useful for determining subsurface structure. We will do our initial analysis in a 2-D sense along the sensor lines and then extend the analysis to 3-D by including correlations between our instrument arrays and the instruments of the Southern California Seismic Network (SCSN), which has approximately 20 permanent stations within and surrounding the basins. We have determined that the correlations can be done over distances up to 40 kilometers and for a frequency range (passband) from 1- to 10-second periods. During the deployment of our SB2, SB3, and SB6 lines, we also installed temporary broadband stations (shown in Figure 1) to supplement the SCSN stations.
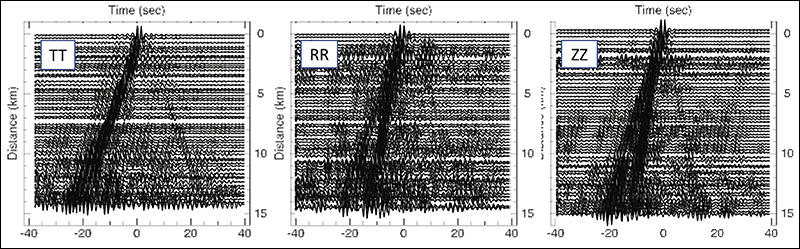
We also plan to try a variety of other techniques to better determine the near-surface structure of the basins as well the deeper structure beneath them, with the primary goal of producing better ground motion predictions for Los Angeles. These techniques include body wave tomography and full-waveform inversion using data from both earthquakes and correlations, horizontal-to-vertical spectral ratios, and autocorrelation imaging. We plan to incorporate the models we determine into the Southern California Earthquake Center’s community velocity models. The data will be available shortly after the final sensor line is completed.
Planning for Resilient Cities
Large earthquakes occurring close to vulnerable metropolitan areas present considerable risk, as demonstrated by the 2010 magnitude 7.0 Haiti earthquake, which devastated the Port-au-Prince metropolitan area [DesRoches et al., 2011]. In California alone, earthquakes cause average annual losses of about $3.7 billion, according to a 2017 report produced by the Federal Emergency Management Agency, the U.S. Geologic Survey, and the Pacific Disaster Center. Losses from the next large earthquake in the state, if it affects a major urban area, are predicted to be even larger [Branum et al., 2016].
Efforts to improve the resilience of cities, so that they are capable of withstanding large earthquakes, are an increasingly essential component of disaster mitigation and urban planning.
Urban, high–seismic hazard settings such as Seattle, Vancouver, Dhaka, and Mexico City [Pagani et al., 2018] that are also, like Los Angeles, underlain by sedimentary basins face additional hidden threats from the loose ground beneath them. In these cases, BASIN-type surveys are essential for obtaining realistic ground motions and accurately assessing seismic hazards and risks.
Urban growth will continue to be the largest contributor to global population increase for the foreseeable future. Thus, efforts to improve the resilience of cities, so that they are capable of withstanding large earthquakes, are an increasingly essential component of disaster mitigation and urban planning [Godschalk, 2003].
Acknowledgments
We are grateful to the deployment and pickup crews from the California State Polytechnic University, Pomona; local California high schools; the Jet Propulsion Laboratory; the California Institute of Technology; and Louisiana State University who helped with the fieldwork; the Los Angeles area homeowners for their willingness to host the nodes; Portable Array Seismic Studies of the Continental Lithosphere (PASSCAL), Louisiana State University, University of Utah, and University of Oklahoma for providing nodes; and the PASSCAL engineers for their help coordinating their nodes. P.P. thanks the Department of Geology and Geophysics at Louisiana State University for supporting this project. This research was partially supported by U.S. Geological Survey awards GS17AP00002 and G19AP00015 and Southern California Earthquake Center awards 18029 and 19033.
References
Bilham, R. (2009), The seismic future of cities, Bull. Earthquake Eng., 7, 839, https://doi.org/10.1007/s10518-009-9147-0.
Branum, D., et al. (2016), Earthquake shaking potential for California, 2016, map sheet, Calif. Dep. of Conserv., Sacramento, www.conservation.ca.gov/cgs/Pages/PSHA/shaking-assessment.aspx.
Denolle, M., et al. (2014), Strong ground motion prediction using virtual earthquakes, Science, 343, 339–403, https://doi.org/10.1126/science.1245678.
DesRoches, R., et al. (2011), Overview of the 2010 Haiti earthquake, Earthquake Spectra, 27(S1), S1–S21, https://doi.org/10.1193/1.3630129.
Godschalk, D. R. (2003), Urban hazard mitigation: Creating resilient cities, Nat. Hazards Rev., 4(3), 136–143, https://doi.org/10.1061/(ASCE)1527-6988(2003)4:3(136).
Graves, R., et al. (2011), CyberShake: A physics-based seismic hazard model for Southern California, Pure Appl. Geophys., 168(3–4), 367–381, https://doi.org/10.1007/s00024-010-0161-6.
Jones, L., et al. (2008), The ShakeOut scenario, U.S. Geol. Surv. Open File Rep., 2008-1150, https://doi.org/10.3133/ofr20081150.
Lin, F.-C., et al. (2013), High-resolution shallow crustal structure in Long Beach, California: Application of ambient noise tomography on a dense seismic array, Geophysics, 78(4), Q45–Q56, https://doi.org/10.1190/geo2012-0453.1.
Liu, G., P. Persaud, and R. Clayton (2018), Structure of the northern Los Angeles basins revealed in teleseismic receiver functions from short-term nodal arrays, Seismol. Res. Lett., 89, 1,680–1,689, https://doi.org/10.1785/0220180071.
Lovely, P., et al. (2006), A structural Vp model of the Salton Trough, California, and its implications for seismic hazard, Bull. Seismol. Soc. Am., 96(5), 1,882–1,896, https://doi.org/10.1785/0120050166.
Olsen, K. B., et al. (2006), Strong shaking in Los Angeles expected from southern San Andreas earthquake, Geophys. Res. Lett., 33, L07305, https://doi.org/10.1029/2005GL025472.
Pagani, M., et al. (2018), Global Earthquake Model (GEM) seismic hazard map, version 2018.1, Dec., Global Earthquake Model Found., Pavia, Italy, https://doi.org/10.13117/GEM-GLOBAL-SEISMIC-HAZARD-MAP-2018.1.
Author Information
Robert W. Clayton ([email protected]), Seismological Laboratory, California Institute of Technology, Pasadena; Patricia Persaud, Department of Geology and Geophysics, Louisiana State University, Baton Rouge; Marine Denolle, Department of Earth and Planetary Sciences, Harvard University, Cambridge, Mass.; and Jascha Polet, Department of Geological Sciences, California State Polytechnic University, Pomona
Citation:
Clayton, R. W.,Persaud, P.,Denolle, M., and Polet, J. (2019), Exposing Los Angeles’s shaky geologic underbelly, Eos, 100, https://doi.org/10.1029/2019EO135099. Published on 10 October 2019.
Text © 2019. The authors. CC BY 3.0
Except where otherwise noted, images are subject to copyright. Any reuse without express permission from the copyright owner is prohibited.