In early August 2019, an Inuit whale hunter working the coastal waters of northwestern Greenland spotted an orange object floating toward the Arctic Ocean. The object turned out to be a lost ocean bottom seismometer (OBS), an instrument typically used to study earthquakes near subduction zones and other seismicity deep below the ocean surface. For me, sitting halfway around the world in Japan, and my colleagues, news of its recovery brought a wave of relief along with reassurance that months of work had not been buried on the seafloor or washed out to sea.
Weeks earlier, we had dropped the instrument several kilometers away into the waters of Bowdoin Fjord, an inlet off the larger, 100-kilometer-long Inglefield Bredning Fjord, to test whether we could make seismic observations amid harsh and noisy polar conditions, a long-standing challenge, and to eavesdrop on nearby Bowdoin Glacier [Podolskiy et al., 2021a]. Marine-terminating, or tidewater, glaciers like Bowdoin crack and split to form crevasses and calve (i.e., produce) icebergs, and meltwater gurgling down the crevasses makes sounds like a bathtub draining. Meanwhile, water flowing beneath glaciers can contribute to seismic tremor, and basal sliding can cause icequakes.
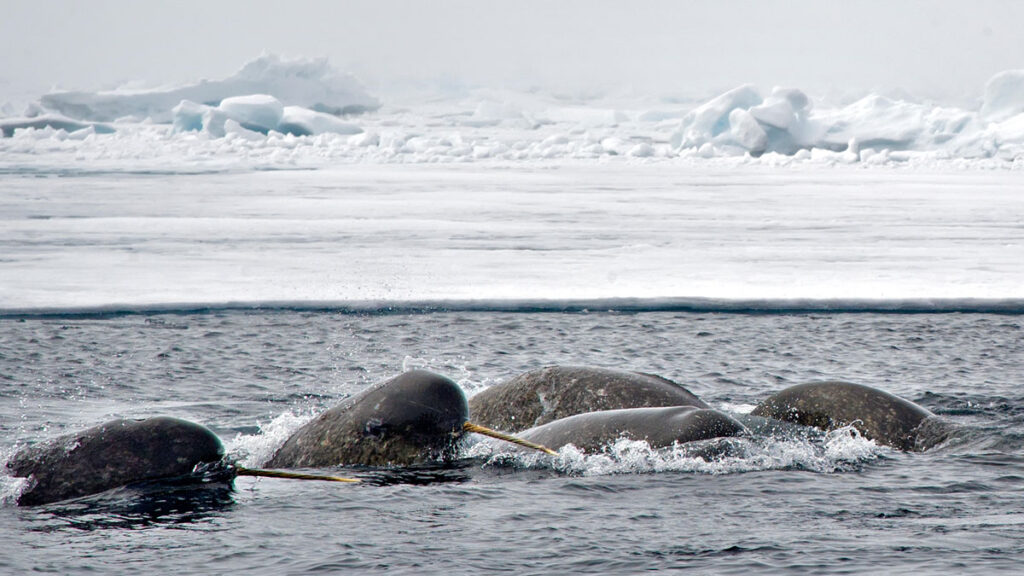
We also wanted to monitor the underwater sounds of glacier fjords and the vocalizations of discreet and shy narwhals—the elusive whales known as “Arctic unicorns” for their extraordinary tusks. For reasons no one fully understands, narwhals, which navigate, communicate, and find food using sound, are sometimes attracted to these noisy environments [Podolskiy and Sugiyama, 2020], although this behavior is not always observed [Heide-Jørgensen et al., 2020]. To record their sounds in hopes of understanding their behavior, we had to get very close, because narwhals’ vocal repertoire often comprises ultrasonic frequencies that are inaudible to humans and attenuate rapidly with distance.
Innovative ways are needed to monitor glaciers, fjords, and endemic wildlife in remote Arctic locations. Such knowledge is important so that we can track and better predict the effects of regional and global climate change. It also may benefit Indigenous Inuit communities who traditionally hunt and subsist on resources provided by narwhals and other fjord-dwelling marine wildlife.
Challenges of Underwater Cryoseismology
Passive seismic monitoring—which involves sensors that receive environmental signals rather than signals from active sources like hammers or explosions—has revolutionized our ability to characterize glaciological processes by recording vibrations from icequakes and tremors [Podolskiy and Walter, 2016]. However, interpreting the data these instruments collect and, indeed, deploying them in glacierized Arctic environments in the first place are not simple tasks.
Strong winds and extensive surface ice cracking make for an extremely noisy seismological environment in which to record data [Podolskiy et al., 2016]. During the Cold War, the United States abandoned its efforts to monitor seismicity from nuclear tests on the Greenlandic coast because the noise from natural causes was so pervasive [Podolskiy et al., 2021b].
Acquiring direct measurements on rapidly changing, fast flowing, calving glaciers involves dangerous fieldwork on heavily crevassed ice in a harsh climate. And there is always the possibility of losing instruments if the ice on which they sit calves into the fjord. Iceberg calving can also make accessing a glacier from the sea dangerous or impossible, although there are sometimes windows of time between major calving events that allow boats or helicopters to approach the calving front for quick operations.
Despite these challenges, the tempting opportunity to monitor an active glacier, as well as recent advancements in deepwater technology and the realization that seafloor seismometers are sensitive to both seismic waves and whale songs [Dréo et al., 2019], gave me the idea to dramatically change our perspective. Rather than placing instruments at only the noisy surface, we could deploy an OBS in the quieter submarine environment far below. From there, we could eavesdrop on sounds coming from the calving front of a marine-terminating glacier—one of the least accessible environments in the cryosphere—and detect who was visiting it.
The plan was to drop a single (very expensive) ocean bottom seismometer (OBS) system in front of an actively calving glacier during a window between major kilometer-scale calving events.
A Warm-Up off Japan
The plan was to drop a single (very expensive) OBS system over the side of a small boat right in front of an actively calving glacier during a window between major kilometer-scale calving events. These events can completely fill the width of a fjord with hundred-meter-wide icebergs and impassable mélange, a floating mixture of smaller icebergs and sea ice.
Not only would there be physical hazards facing this deployment, but also there was the added complication that I—a glaciologist more comfortable working on ice who always gets seasick on research vessels—would be leading a team of colleagues who, like me, had little experience working with OBSs. All this added up to the need for a practice run of sorts, so that I could learn how to assemble and operate an OBS on my own in difficult conditions.
The only way to learn the ins and outs of OBS preparation, deployment, and retrieval methods was to do it firsthand, an opportunity I got thanks to Yoshio Murai, a marine seismologist at Hokkaido University. Murai invited me to participate in a research cruise focused on studying the locked segment of the subduction zone off eastern Hokkaido Island, near Kushiro, Japan, which has the potential to generate major earthquakes with accompanying tsunamis.
In spring 2019, after assembling 10 OBS instruments in the laboratory, we deployed them—worth about half a million dollars in total—from a large research vessel to depths of up to 3 kilometers in the Pacific Ocean, with the support of technicians operating a crane. Three months later, we retrieved the OBSs by sending the instruments an acoustic command to release their anchoring ballasts and float to the surface.
Soon after my successful warm-up run at Hokkaido, we sent a single OBS system—comprising seismic and other sensors—to Greenland for the pilot test in Bowdoin Fjord, a key summering ground for narwhals [Podolskiy and Sugiyama, 2020]. This time, however, I did not have an expert with 2 decades of OBS experience by my side or a research vessel, a crane, or even a proper port to undertake the task.
Taking the Plunge in Greenland
In July 2019, I set up an improvised workshop in the living room of an Inuit hunter’s summer house in Qaanaaq, a settlement in northwestern Greenland with roughly 600 inhabitants. There, “on the edge of the livable world” at 77.46°N as Hastrup [2019] has described it, I assembled the internal components of the OBS—the seismometer itself and the data recorder, batteries, and wiring—and vacuum-sealed them in a protected glass sphere.
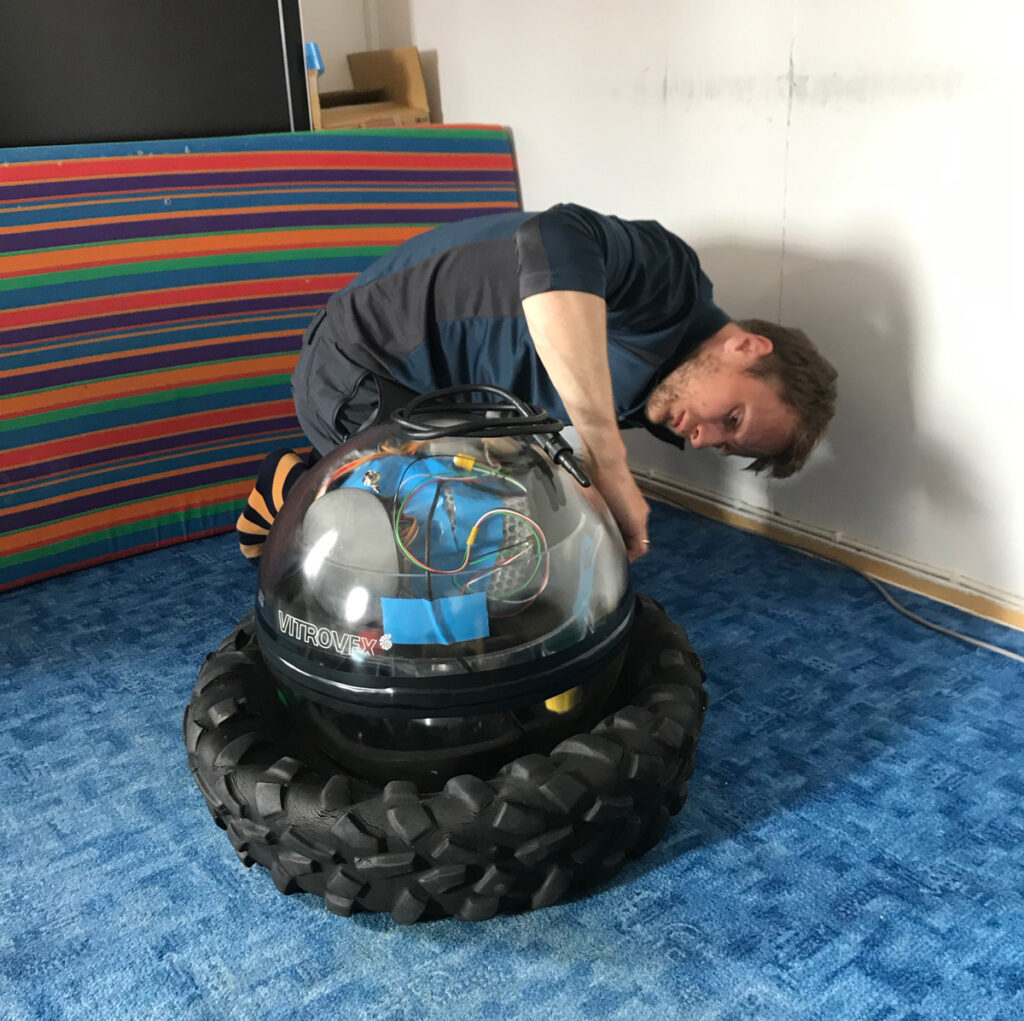
On 21 July, my colleagues and I used a truck and a tiny inflatable raft to move all our equipment piece by piece from Qaanaaq to a small boat, which we motored to a small, rock-walled bay in 20-kilometer-long Bowdoin Fjord. It was the same bay where explorer Robert Peary anchored the Falcon in 1893. During that expedition nearly 130 years ago, Peary and his crew collected observations that document that Bowdoin Glacier was then 3 kilometers longer than it is today. In summer 2019, as we sheltered in the bay, which was still bloodstained from the day before when local hunters had butchered a narwhal and seals, I made final preparations before the deployment, installing a high-frequency hydrophone and a thermometer for recording narwhal vocalizations and water temperature. All our eggs were now in one basket, so to speak.
Finally, my colleagues, our local guides, and I reached the middle of the fjord, several hundred meters from the glacier’s calving front. Here capsizing icebergs generate meters-high tsunamis much more frequently than they occur in any subduction zone, so we had to work quickly to reduce our risk of capsizing or being swept away.
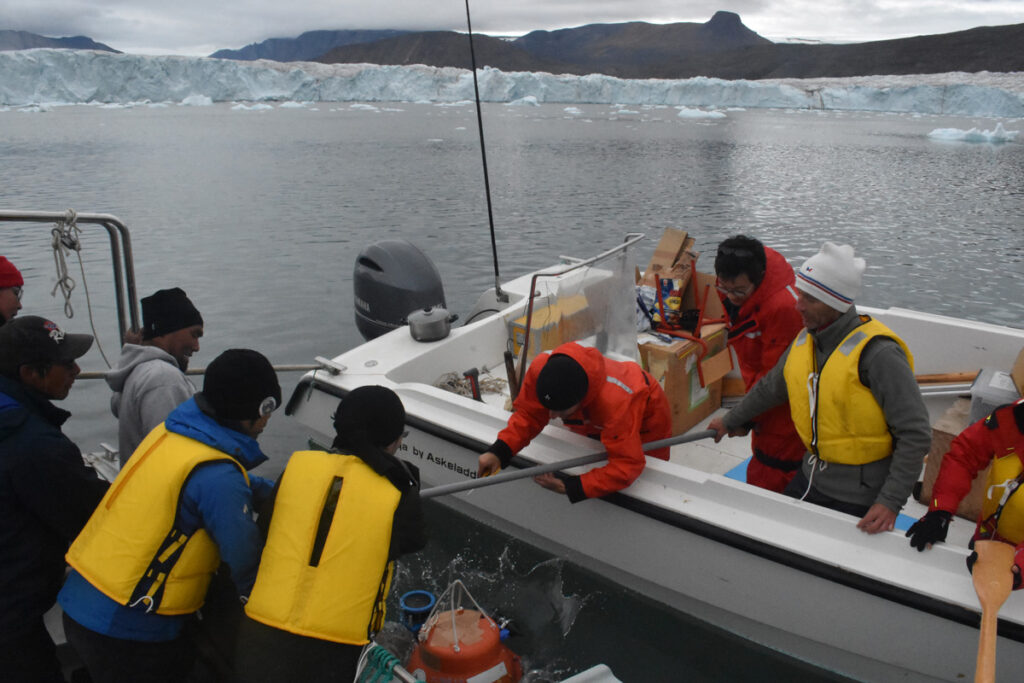
Without a crane, we tied the OBS with rope to a metal pole held between two boats to hoist the delicate system over the water without damaging the sensitive ballast release mechanism. I then cut the rope, and the heavy instrument, together with half a year of preparations, plunged into the water. It reached the bottom of the fjord 243 meters below about 3 minutes later, ready to begin collecting data. At this depth, we assumed the OBS would be safe from the monster icebergs that occasionally calved into the fjord until we could retrieve it a couple of weeks later.
Lost and Found
Because of unforeseen delays, including a major calving event on 29 July that blocked access to the fjord for days, I was unable to visit it to “call” the instrument back to the surface before my flight out of Greenland. I asked a colleague, Naoya Kanna, an oceanographer at the University of Tokyo who is experienced in mooring deployments, to do the honors, knowing the OBS retrieval was in good hands. On 6 August, however, the long-awaited morning of the retrieval, as I made my morning coffee at home back in Japan before my kids woke up, I opened an email from Naoya that read, “I sent the ‘release’ command to your OBS, but it never came up.”
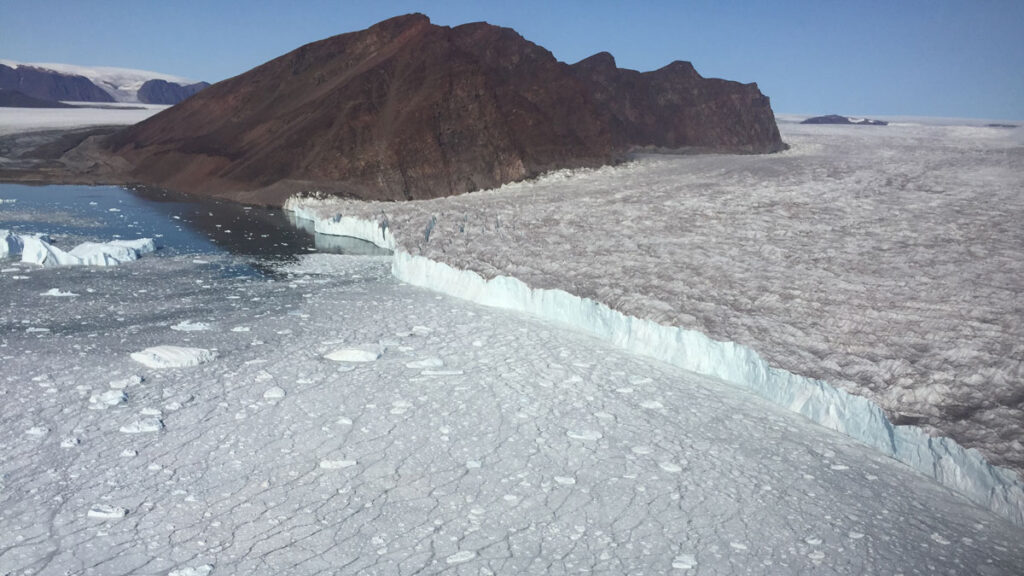
After a satellite phone call to follow up, it was clear that something had gone wrong. Did I fail to prepare the instrument correctly? Did a massive iceberg scrape it off the ocean bottom after all, or did icebergs passing above deposit rock debris that buried the instrument? Did curious seals damage the cables? I thought I might never know. My coffee was no longer satisfying after such an extreme disappointment.
Three days later, I received another, far more welcome message from Naoya: “I am happy to tell you that a hunter found your OBS in the fjord.”
An Inuit hunter, Rusmus Daorana, found our wayward instrument floating about 7 kilometers from the deployment location and left it on the coast, where Naoya found it—coated in fine glacial till—and retrieved it. The hard drives from the OBS reached me a month later, revealing that the instrument had returned to the surface about 10 hours after Naoya sent the release command. Our best guess is that the OBS sank into soft sediments (hence the till) at the bottom of the fjord, where sediment from glacial runoff accumulates very rapidly, which slowed the release mechanism. Clearly, this is a factor we need to consider in future experiments [Podolskiy et al., 2021a].
Surveying an Arctic Soundscape
All told, the hard drives contained 432 gigabytes of continuous seismoacoustic signals from icequakes, narwhals, and other exciting phenomena. While the OBS was collecting data, an instrumented mooring 1 kilometer from the glacial terminus recorded a spike in the speed of the water current flowing out of the fjord. This spike coincided with the 29 July calving event, which was recorded in our seismoacoustic data and would have displaced a large amount of water toward the mooring. These data also represented the first underwater recording—and the closest recording of any kind to date—of a powerful glacial earthquake, which was produced by the calving event and was seismically detectable 500 kilometers away [Podolskiy et al., 2021a, 2021b].
The OBS temperature record, meanwhile, indicated dropping deepwater temperatures at the fjord floor over the duration of the deployment, consistent with an ongoing extreme melt event at the glacier’s surface amid abnormally high air temperatures. This drop suggested that the input of fresh and relatively light glacial meltwater had induced a change in the stratification of the water in the fjord [Podolskiy et al., 2021a]. Among other effects, such shifts may influence melting along the ice cliff as well as the biogeochemistry of fjord waters.
The ambient seismic noise recorded by our OBS showed that these instruments can detect continuous seismic vibrations radiating from a glacier sliding against the rock below.
Our analysis of the ambient seismic noise recorded by our OBS showed that these instruments can detect continuous seismic vibrations, reminiscent of those from slow earthquakes, radiating from a glacier sliding against the rock below [Podolskiy et al., 2021b]. This information may tell us about a glacier’s speed and help to constrain conditions—such as friction and the effective pressure of the overlying ice—at its base. The conditions of basal sliding at fast moving glaciers, which have important implications for projections of ice discharge and sea level, have long been unclear.
It is nearly impossible to study these conditions directly, which would require hazardous drilling near the crevassed glacial terminus. Drone mapping and GPS and radar observations, meanwhile, are collected over shorter time periods, are exposed to hazards at the surface, and require a lot of effort by operators. The ability of an underwater seismometer to hear how fast a glacier slides offers a novel way to collect continuous information about glacier motion in the noisy and harsh polar environment. Not only that, but OBSs may offer a means of detecting whether a tidewater glacier is detaching from its rocky base and becoming a floating ice shelf, a transition that affects glacial dynamics and retreat rates. This is a possibility to explore with further deployments.
It has long been known that glacial calving makes a great deal of noise underwater (although this noise has likely increased recently as glacial earthquakes have become more frequent than they were several decades ago). Indeed, our analyses of the recordings from the major July calving event suggest that calving produces some of the loudest sounds in the Arctic Ocean, like underwater explosions of several tons of TNT [Podolskiy et al., 2021c]. I wondered what impacts such loud sounds might have on marine mammals. From our visual observations from the boats, we knew there were mammals, including narwhals and seals, in the fjord during our campaign, but were they near the glacier during that 30-minute calving event?
In the seismoacoustic data, at the beginning of the calving event, I found tiny, but characteristic, ultrasound clicks and buzzes produced by narwhals swimming very close to the calving front. Following technical guidance from the U.S. National Marine Fisheries Service on estimating the risk of auditory injury in marine mammals, we found that glacial calving sounds are so loud that they might damage the hearing of narwhals and seals [Podolskiy et al., 2021c]. This observation further highlights that an OBS can be a truly multipurpose tool in glacial settings.
Much More to Learn
For all the interesting observations we collected from the bottom of Bowdoin Fjord in 2019, many new questions have arisen, and others remain unanswered. For example, despite our idea that the intense noise produced by calving glaciers and capsizing icebergs could harm narwhals and seals, these animals’ resilience to noise exposure is still unclear. The effects of anthropogenic underwater noise, such as that from seismic air guns, on marine animals have been extensively discussed [Protection of the Arctic Marine Environment, 2019], but no studies have focused on loud noises from icebergs and glaciers.
Why are narwhals attracted to the dangerous calving front in the first place? It is known that subglacial meltwater plumes attract foraging birds and seals [Lydersen et al., 2014] and that prey for narwhals—like Arctic cod, Greenland halibut, and other fish—inhabit glacial fjords in this region. However, some studies have suggested that narwhals do not forage much in summer, so their attraction to this noisy region at this time of year remains an enigma [e.g., Podolskiy and Sugiyama, 2020].
Conducting cryoseismology underwater for the first time was not easy, but our experience demonstrated the tremendous potential of the approach.
With respect to glaciers, our data showed continuous seismic tremor, indicative of basal sliding, in summer, but does this seismic rumble also occur in winter? And does it occur at other glaciers? If so, what does this behavior tell us about how these glaciers are being affected by climate change? Also, can we detect noise from subglacial meltwater discharge and possibly determine the volume of runoff by analyzing this noise? Similarly, can we detect noise with an OBS from an ice cliff melting below the water as pressurized air bubbles are released [e.g., Deane et al., 2019]?
These are all questions we are looking to address further with the limited amount of data collected during our 2019 deployment, as well as with additional data from future deployments. Ultimately, we hope to develop a long-term underwater monitoring network in the area and to integrate it with complementary multipurpose campaigns (e.g., for glacier monitoring and animal sightings).
Conducting cryoseismology underwater for the first time was not easy, but our experience demonstrated the tremendous potential of the approach in helping us better understand processes and changes occurring in glacial fjords and the behavior of fjord inhabitants. These data should help scientists trying to piece together influences of tidewater glaciers on marine conditions and sea level rise, as well as Arctic communities for whom knowledge of local environments and wildlife is vital to sustaining their ways of life.
Acknowledgments
This study was possible due to financial support from the Arctic Challenge for Sustainability research projects (ArCS and ArCS II) and Grants-in-Aid for Scientific Research “KAKENHI” 18K18175. The Ministry for Nature, Environment and Justice (government of Greenland) granted permission (C-19-37) to conduct this fieldwork in Greenland. I appreciate discussion with G. Barruol (Institut de Physique du Globe de Paris, IPGP) on OBS observations. I am also incredibly thankful for the significant support I have received from my colleagues Y. Murai, S. Sugiyama, I. Asaji, T. Ando, and Y. Sakuragi (Hokkaido University); N. Kanna (University of Tokyo); A. Mangeney (IPGP); and O. Castelnau (Centre National de la Recherche Scientifique), from our local guides (T. Oshima, K. Petersen, Q. Aladaq, and S. Aladaq), and from the hunter (R. Daorana) who found our OBS floating not so far from the North Pole.
References
Deane, G. B., et al. (2019), The underwater sounds of glaciers, Acoust. Today, 15(4), 12–19, https://doi.org/10.1121/AT.2019.15.4.12.
Dréo, R., et al. (2019), Baleen whale distribution and seasonal occurrence revealed by an ocean bottom seismometer network in the western Indian Ocean, Deep Sea Res., Part II, 161, 132–144, http://dx.doi.org/10.1016/j.dsr2.2018.04.005.
Hastrup, K. (2019), The historicity of health: Environmental hazards and epidemics in northwest Greenland, Cross Cult. Res., 53(3), 291–311, https://doi.org/10.1177%2F1069397118806823.
Heide-Jørgensen, M. P., et al. (2020), Some like it cold: Temperature-dependent habitat selection by narwhals, Ecol. Evol., 10(15), 8,073–8,090, https://doi.org/10.1002/ece3.6464.
Lydersen, C., et al. (2014), The importance of tidewater glaciers for marine mammals and seabirds in Svalbard, Norway, J. Mar. Syst., 129, 452–471, https://doi.org/10.1016/j.jmarsys.2013.09.006.
Podolskiy, E. A., and F. Walter (2016), Cryoseismology, Rev. Geophys., 54, 708–758, https://doi.org/10.1002/2016RG000526.
Podolskiy, E. A., and S. Sugiyama (2020), Soundscape of a narwhal summering ground in a glacier fjord (Inglefield Bredning, Greenland), J. Geophys. Res. Oceans, 125, e2020JC016116, https://doi.org/10.1029/2020JC016116.
Podolskiy, E. A., et al. (2016), Tide-modulated ice flow variations drive seismicity near the calving front of Bowdoin Glacier, Greenland, Geophys. Res. Lett., 43, 2,036–2,044, https://doi.org/10.1002/2016GL067743.
Podolskiy, E. A., et al. (2021a), Ocean-bottom seismology of glacial earthquakes: The concept, lessons learned, and mind the sediments, Seismol. Res. Lett., 92(5), 2,850–2,865, https://doi.org/10.1785/0220200465.
Podolskiy, E. A., et al. (2021b), Ocean-bottom and surface seismometers reveal continuous glacial tremor and slip, Nat. Commun., 12, 3929, https://doi.org/10.1038/s41467-021-24142-4.
Podolskiy, E. A., et al. (2021c), Glacial earthquake-generating iceberg calving in a narwhal summering ground: The loudest underwater sound in the Arctic? J. Acoust. Soc. Am., accepted for publication.
Protection of the Arctic Marine Environment (2019), Underwater noise in the Arctic: A state of knowledge report, Arctic Counc. Secr., Tromsø, Norway, oaarchive.arctic-council.org/handle/11374/2394.
Author Information
Evgeny A. Podolskiy ([email protected]), Arctic Research Center, Hokkaido University, Sapporo, Japan