Imagine it’s 3:00 a.m. along the Pacific Northwest coast—it’s dark outside and most people are asleep indoors rather than alert and going about their day. Suddenly, multiple seismometers along the coast of Washington state are triggered as seismic waves emanate from a seconds-old earthquake. These initial detections are followed rapidly by subsequent triggering of a dozen more instruments spread out both to the north, toward Seattle, and to the south, toward Portland, Ore. Across the region, as the ground begins to shake and windows rattle or objects fall from shelves, many people wake from sleep—while others are slower to sense the potential danger.
Within a few seconds of the seismometers being triggered, computers running long-practiced seismic location and magnitude algorithms estimate the source of the shaking: a magnitude 7.0 earthquake 60 kilometers off the Washington coast at a depth roughly consistent with the Cascadia Subduction Zone (CSZ) interface, along which one tectonic plate scrapes—and occasionally lurches—past another as it descends toward Earth’s interior. The CSZ is a well-studied fault known in the past to have produced both magnitude 9 earthquakes and large tsunamis—the last one in 1700.
Although a magnitude 7 quake on the Cascadia Subduction Zone could certainly cause damage, a magnitude 8 or 9 quake would shake a vastly larger region and could produce devastating tsunamis that would inundate long stretches of coastline.
The initial information provided by seismometers is important in alerting not only scientists but also emergency response personnel and the public to the potentially hazardous seismic activity. But whether these early incoming seismic waves truly represent a magnitude 7 event, whose causative fault ruptured for 15–20 seconds, or whether instead they reflect ongoing fault slip that could last minutes and spread hundreds of kilometers along the fault—representing a magnitude 8 or even 9 earthquake—is very difficult to discern in real time using only local seismometers.
It’s a vital distinction: Although a magnitude 7 quake on the CSZ could certainly cause damage, a magnitude 8 or 9 quake—potentially releasing hundreds of times more energy—would shake a vastly larger region and could produce devastating tsunamis that would inundate long stretches of coastline. Some communities must evacuate for miles to get out of the potential inundation zone, meaning that every second counts. The ability to characterize earthquake slip and location accurately within a minute or two of a fault rupturing controls how effective early warnings are and could thus mean the difference between life and death for tens of thousands of people living today along the Pacific Northwest coast.
Enter GPS or, more generally, Global Navigation Satellite Systems (GNSS). These systems comprise constellations of Earth-orbiting satellites whose signals are recorded by receivers on the ground and used to determine the receivers’ precise locations through time. GPS is the U.S. system, but several countries, or groups of countries, also operate independent GNSS constellations, including Russia’s GLONASS and the European Union’s Galileo system, among others. Prominently used for navigational purposes, GNSS ground receivers, which in recent years have proliferated by the thousands around the world, now offer useful tools for rapidly and accurately characterizing large earthquakes—supplementing traditional seismic detection networks—as well as many other natural hazards.
An Initial Demonstration
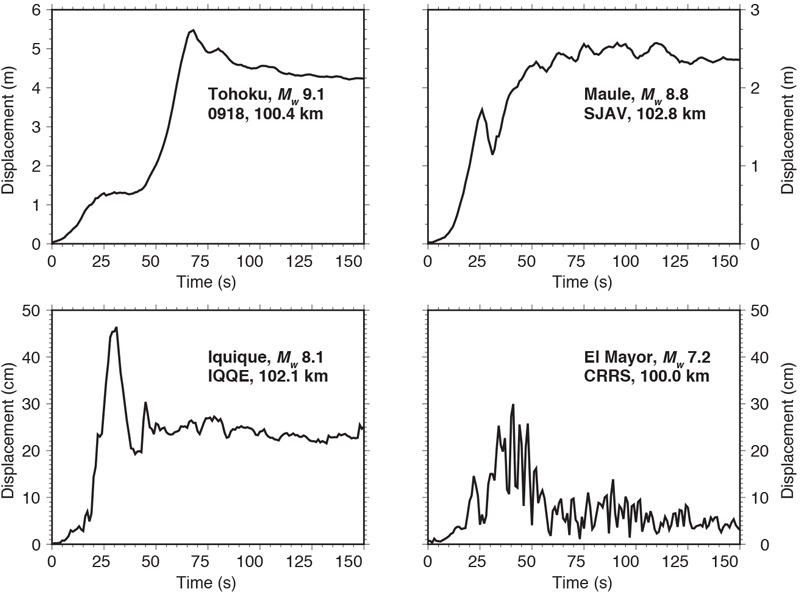
Large earthquakes both strongly shake and deform the region around the source fault to extents that GNSS can easily resolve (Figure 1). With the expansion of GNSS networks and continuous telemetry, seismic monitoring based on GNSS measurements has come online over the past few years, using continuously gathered position data from more than a thousand ground stations, a number that is steadily growing. Station positions are computed in a global reference frame at an accuracy of a few centimeters within 1–2 seconds of data acquisition in the field. In the United States, these data are fed into U.S. Geological Survey (USGS) and National Oceanic and Atmospheric Administration (NOAA) centers charged with generating and issuing earthquake and tsunami early warnings.
In the scenario above, GNSS-based monitoring would provide an immediate discriminant of earthquake size based on the amount of displacement along the coast of Washington state. Were it a magnitude 7, a dozen or so GNSS stations spread along a roughly 30-kilometer span of the coast might reasonably move a few tens of centimeters within half a minute, whereas a magnitude 8 event—or a magnitude 9 “full rip” along the entire subduction zone, from California to British Columbia—would move hundreds of Cascadia GNSS stations many meters. Ground offset at some might exceed 10 meters, depending on location, but the timing of the offsets along the coast determined with GNSS would track the rupture itself.
The July 2019 strike-slip earthquake sequence in the Eastern California Shear Zone near Ridgecrest in the eastern Mojave Desert provided the first real-world demonstration of the capability of GNSS-based seismic monitoring. The newly developed GNSS monitoring systems included a dozen GNSS stations from the National Science Foundation–supported Network of the Americas (NOTA) located near the fault rupture. Data from these stations indicated that the magnitude 7.1 main shock on 5 July caused coseismic offsets of up to 70 centimeters in under 30 seconds of the initiation of fault slip.
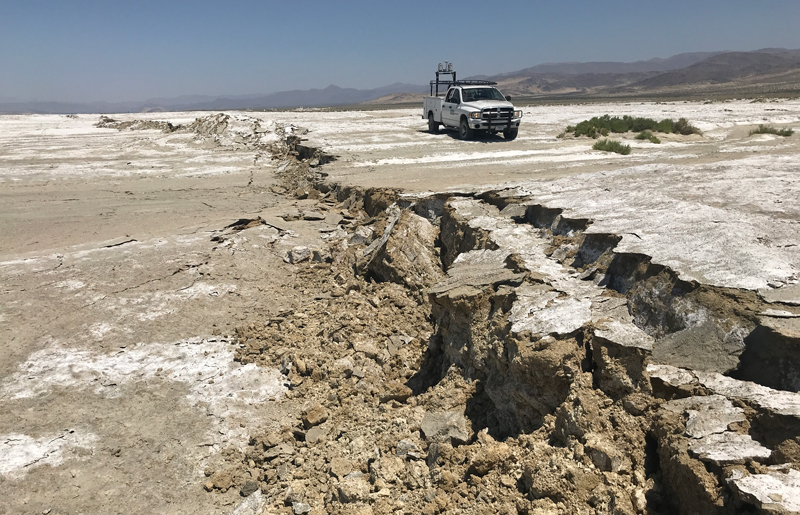
Further analysis of the data showed that those 30 seconds encompassed the fault rupture duration itself (roughly 10 seconds), another 10 or so seconds as seismic waves and displacements propagated from the fault rupture to nearby GNSS stations, and another few seconds for surface waves and other crustal reverberations to dissipate sufficiently such that coseismic offsets could be cleanly estimated. Latency between the time of data acquisition in the Mojave Desert to their arrival and processing for position at Central Washington University was less than 1.5 seconds, a fraction of the fault rupture time itself. Comparison of the coseismic ground deformation estimated within 30 seconds of the event with that determined several days later, using improved GNSS orbital estimates and a longer data window, shows that the real-time offsets were accurate to within 10% of the postprocessed “true” offsets estimated from daily positions [Melgar et al., 2019]. Much of the discrepancy may be attributable to rapid fault creep in the hours after the earthquake.
A Vital Addition for Hazards Monitoring
This new ability to accurately gauge the position of GNSS receivers within 1–2 seconds from anywhere on Earth has opened a new analysis pipeline that remedies known challenges for our existing arsenal of monitoring tools. Receiver position data streams, coupled to existing geophysical algorithms, allow earthquake magnitudes to be quickly ascertained via simple displacement scaling relationships [Crowell et al., 2013]. Detailed information about fault orientation and slip extent and distribution can also be mapped nearly in real time as a fault ruptures [Minson et al., 2014]. These capabilities may prove particularly useful for earthquake early warning systems: GNSS can be incorporated into these systems to rapidly constrain earthquake magnitude, which determines the areal extent over which warnings are issued for a given shaking intensity [Ruhl et al., 2017].
For large earthquakes, GNSS will likely guide the issuance of rapid-fire revised warnings as a rupture continues to grow throughout and beyond the timing of initial, seismometer-based characterization.
GNSS will never replace seismometers for immediate earthquake identifications because of its vastly lower sensitivity to small ground displacements. But for large earthquakes, GNSS will likely guide the issuance of rapid-fire revised warnings as a rupture continues to grow throughout and beyond the timing of initial, seismometer-based characterization [Murray et al., 2019].
Deformation measured using GNSS is also useful in characterizing tsunamis produced by earthquakes, 80% of which in the past century were excited either by direct seismic uplift or subsidence of the ocean floor along thrust and extensional faults [Kong et al., 2015] or by undersea landslides, such as in the 2018 Palu, Indonesia, earthquake (A. Williamson et al., Coseismic or landslide? The source of the 2018 Palu tsunami, EarthArXiv, https://doi.org/10.31223/osf.io/fnz9j). Rough estimates of tsunami height may be computed nearly simultaneously with fault slip by combining equations describing known hydrodynamic behavior with seafloor uplift determined from GNSS offsets [Melgar et al., 2016]. Although GNSS won’t capture landslides or other offshore processes for which on-land GNSS has little resolution, the rapidity of the method in characterizing tsunami excitation, compared with the 10–20 minutes required by global tide gauge and seismic networks and by NOAA’s tsunami-specific Deep-Ocean Assessment and Reporting of Tsunamis (DART) buoy system, offers a dramatic potential improvement in response time for local tsunamis that can inundate coastlines within 5–15 minutes of an earthquake.
Natural hazards monitoring using GNSS isn’t limited to just solid Earth processes. Other measurable quantities, such as tropospheric water content, are estimated in real time with GNSS and are now being used to constrain short-term weather forecasts. Likewise, real-time estimates of ionospheric electron content from GNSS can help identify ionospheric storms (space weather) and in mapping tsunami-excited gravity waves in the ionosphere to provide a more direct measurement of the propagating tsunami as it crosses oceanic basins.
A Future of Unimaginable Potential
Many resources beyond the rapid proliferation of GNSS networks themselves have contributed to making global GNSS hazards monitoring a reality. Unlike seismic sensors that measure ground accelerations or velocities directly, GNSS positioning relies on high-accuracy corrections to the orbits and clocks broadcast by satellites. These corrections are derived from continuous analyses of global networks of ground stations. Similarly, declining costs of continuous telemetry have facilitated multiconstellation GNSS processing, using the vast investments in international satellite constellations to further improve the precision and reliability of real-time GNSS measurements of ground displacements.
Throughout the seismically active Americas, from Alaska to Patagonia, numerous GNSS networks now operate, leaving big earthquakes without many places to hide.
In the future, few large earthquakes in the western United States will escape nearly instantaneous measurement by real-time GNSS. Throughout the seismically active Americas, from Alaska to Patagonia, numerous GNSS networks in addition to NOTA now operate, leaving big earthquakes without many places to hide. Mexico operates several GNSS networks, as do Central and South American nations from Nicaragua to Chile. Around the Pacific Rim, Japan, New Zealand, Australia, and Indonesia all operate networks that together comprise thousands of ground stations.
In North America, nearly all GNSS networks have open data-sharing policies [Murray et al., 2018]. But a global system for hazard mitigation can be effective only if real-time data are shared among a wider set of networks and nations. The biggest remaining impediment to expanding a global system is increasing the networks whose data are available for monitoring. GNSS networks are expensive to deploy and maintain. Many networks are built in whole or in part for land surveying and operate in a cost-recovery mode that generates revenue by selling data or derived positioning corrections through subscriptions. At the current time, just under 3,000 stations are publicly available for hazards monitoring, but efforts are under way to create international data sharing agreements specifically for hazard reduction. The Sendai Framework for Disaster Risk Reduction, administered by the United Nations Office for Disaster Risk Reduction, promotes open data for hazard mitigation [International Union of Geodesy and Geophysics, 2015], while professional organizations, such as the International Union of Geodesy and Geophysics, promote their use for tsunami hazard mitigation [LaBrecque et al., 2019].
The future holds unimaginable potential. In addition to expanding GNSS networks, modern smartphones by the billions are ubiquitous sensing platforms with real-time telemetry that increasingly make many of the same GNSS measurements that dedicated GNSS receivers do. Crowdsourcing, while not yet widely implemented, is one path forward that could use tens of millions of phones, coupled to machine learning methods, to help fill in gaps in ground displacement measurements between traditional sensors.
The potential of GNSS as an important supplement to existing methods for real-time hazards monitoring has long been touted. However, a full real-world test and demonstration of this capability did not occur until the recent Ridgecrest earthquake sequence. Analyses are ongoing, but so far the conclusion is that the technique performed exactly as expected—which is to say, it worked exceedingly well. GNSS-based hazards monitoring has indeed arrived.
Acknowledgments
Development of global GNSS seismic analysis is supported by NASA-ESI grants NNX14AQ40G and 80NSSC19K0359 and USGS Cooperative Agreements G17AC00344 and G19AC00264 to Central Washington University. Data from the Network of the Americas are provided by the Geodetic Facility for the Advancement of Geoscience (GAGE), operated by UNAVCO Inc., with support from the National Science Foundation and NASA under NSF Cooperative Agreement EAR-1724794.
References
Crowell, B. W., et al. (2013), Earthquake magnitude scaling using seismogeodetic data, Geophys. Res. Lett., 40(23), 6,089–6,094, https://doi.org/10.1002/2013GL058391.
International Union of Geodesy and Geophysics (2015), Resolution 4: Real-time GNSS augmentation of the tsunami early warning system, iugg.org/resolutions/IUGGResolutions2015.pdf.
Kong, L. S. L., et al. (2015), Pacific Tsunami Warning System: A Half-Century of Protecting the Pacific 1965–2015, 188 pp., Int. Tsunami Inf. Cent., Honolulu, Hawaii, unesdoc.unesco.org/ark:/48223/pf0000233564.
LaBrecque, J., J. B. Rundle, and G. W. Bawden (2019), Global navigation satellite system enhancement for tsunami early warning systems, in Global Assessment Report on Disaster Risk Reduction, U.N. Off. for Disaster Risk Reduct., Geneva, Switzerland, unisdr.org/files/66779_flabrequeglobalnavigationsatellites.pdf.
Melgar, D., et al. (2016), Local tsunami warnings: Perspectives from recent large events, Geophys. Res. Lett., 43(3), 1,109–1,117, https://doi.org/10.1002/2015GL067100.
Melgar, D., et al. (2019), Real-time high-rate GNSS displacements: Performance demonstration during the 2019 Ridgecrest, CA earthquakes, Seismol. Res. Lett., in press.
Minson, S. E., et al. (2014), Real-time inversions for finite fault slip models and rupture geometry based on high-rate GPS data, J. Geophys. Res. Solid Earth, 119(4), 3,201–3,231, https://doi.org/10.1002/2013JB010622.
Murray, J. R., et al. (2018), Development of a geodetic component for the U.S. West Coast Earthquake Early Warning System, Seismol. Res. Lett., 89(6), 2,322–2,336, https://doi.org/10.1785/0220180162.
Murray, J. R., et al. (2019), Regional Global Navigation Satellite System networks for crustal deformation monitoring, Seismol. Res. Lett., https://doi.org/10.1785/0220190113.
Ruhl, C. J., et al. (2017), The value of real-time GNSS to earthquake early warning, Geophys. Res. Lett., 44(16), 8,311–8,319, https://doi.org/10.1002/2017GL074502.
Ruhl, C. J., et al. (2019), A global database of strong-motion displacement GNSS recordings and an example application to PGD scaling, Seismol. Res. Lett., 90(1), 271–279, https://doi.org/10.1785/0220180177.
Author Information
Timothy I. Melbourne ([email protected]), Pacific Northwest Geodetic Array, Department of Geological Sciences, Central Washington University, Ellensburg; Diego Melgar, Department of Geological Sciences, University of Oregon, Eugene; Brendan W. Crowell, Department of Earth and Space Sciences, University of Washington, Seattle; and Walter M. Szeliga, Pacific Northwest Geodetic Array, Department of Geological Sciences, Central Washington University, Ellensburg
Citation:
Melbourne, T. I.,Melgar, D.,Crowell, B. W., and Szeliga, W. M. (2019), Seismic sensors in orbit, Eos, 90, https://doi.org/10.1029/2019EO138001. Published on 26 December 2019.
Text © 2019. The authors. CC BY-NC-ND 3.0
Except where otherwise noted, images are subject to copyright. Any reuse without express permission from the copyright owner is prohibited.